Page snapshot: Introduction to energy, including definition of energy and units used to measure energy, fossil fuel types and their extraction, renewable energy, and the future of energy in the United States.
Topics covered on this page: What is energy?; What do different units of energy mean?; How do we look at energy in the Earth system?; Becoming "energy literate"; Fossil fuels; Oil and gas; Oil drilling; Coal; Coalbed methane; Renewable energy; Bioethanol and biomass plants; Geothermal; Hydroelectric; Wind; The future of energy in the US; Adaptation; Resources.
Credits: Most of the text of this page is derived from "Energy in the Southeastern US" by Carlyn S. Buckler, Peter L. Nester, Stephen F Greb, and Robert J. Moye, chapter 6 in The Teacher-Friendly Guide to the Earth Science of the Southeastern U.S., 2nd. ed., edited by Andrielle N. Swaby, Mark D. Lucas, and Robert M. Ross (published in 2016 by the Paleontological Research Institution; currently out of print), with some text coming from other volumes of the Teacher-Friendly Guide series. The book was adapted for the web by Elizabeth J. Hermsen and Jonathan R. Hendricks in 2021–2022. Changes include formatting and revisions to the text and images. Credits for individual images are given in figure captions.
Updates: Page last updated April 7, 2022.
Image above: An aerial photograph of energy production infrastructure in the Williston Basin, Montana. Source: USGS (public domain).
What is energy?
Energy is an interdisciplinary topic, and the concepts used to understand energy in the Earth system are fundamental to all disciplines of science. One cannot study physics or understand biomes, photosynthesis, fire, evolution, seismology, chemical reactions, or genetics without considering energy. Everything we do depends upon energy—without it there would be no civilization, no sunlight, no food, and no life. Energy moves people and goods, produces electricity, and heats our homes and businesses. It is used in manufacturing and other industrial processes.
But what is energy? Energy is power that is derived from the utilization of physical or chemical resources. Wind and solar power, fossil fuels, nuclear energy, and hydroelectricity are primary energy sources. Primary energy sources are energy sources that occur in nature. Secondary energy sources, also known as energy carriers, have been transformed into energy used directly by humans. Examples of secondary energy sources are electricity and gasoline.
For most of human history, the way we captured and used energy changed little. With very few exceptions, materials were moved by human or animal power. Heat was produced largely through the burning of wood. Exceptions include the use of sails on boats by a very small percentage of the world’s population to move people and goods. In China, people used natural gas to boil brine in the production of salt beginning roughly 2000 years ago. Nearly all the energy to power human society was, in other words, biomass; it was produced by humans, by other animals, or by burning wood.
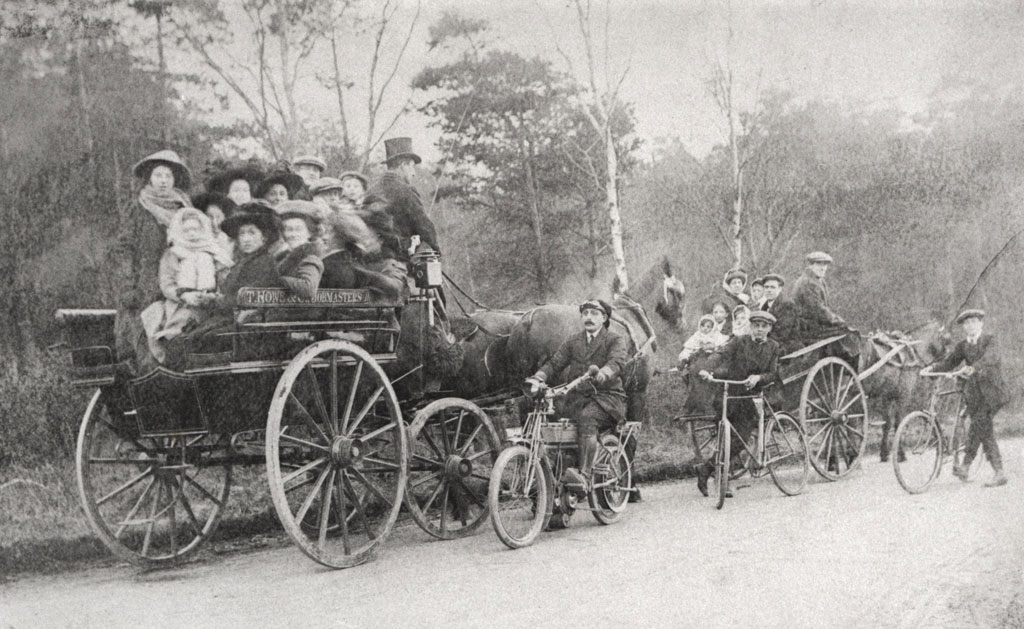
People traveling by bicycle and horse-drawn carriage, Newcastle-upon-Tyne, England, 1910. In this picture, all the energy for transport is being produced by humans or horses. Photo from Newcastle Libraries (059169, flickr, public domain).
The transition from brute force and burning wood to the production and use of various industrial sources of energy has occurred remarkably quickly, happening in the course of just a few generations. Much of the rural US was without access to electricity until the 1930s, and cars have been around for only slightly longer. Yet, many of us take these conveniences for granted today. The transition to industrial sources of energy has caused changes in virtually every aspect of human life, from transportation to economics to war to architecture. In the US, every successive generation has enjoyed the luxury of more advanced technology (e.g., the ability to travel more frequently, more quickly, and over greater distances). Especially as the global population grows and standards of living increase in some parts of the world, so too does global energy demand continue to grow.
Our energy system—how we get energy and what we use it for—is still changing remarkably quickly in some ways, while it is very resistant to change in others. The use of wind to generate electricity, for example, grew rapidly in the late 2000s and early 2010s. In 2002, wind produced less than 11 million megawatt hours (MWh) of electricity in the US, whereas in 2011, wind produced more than 120 million MWh. In contrast, we continue to rely heavily on fossil fuels like coal, oil, and natural gas to produce electricity, supply heat, and fuel transportation. Our reliance on fossil fuels is driven by a number of factors, including low upfront cost, very high energy densities, and the cost and durability of the infrastructure built to use fossil fuels.
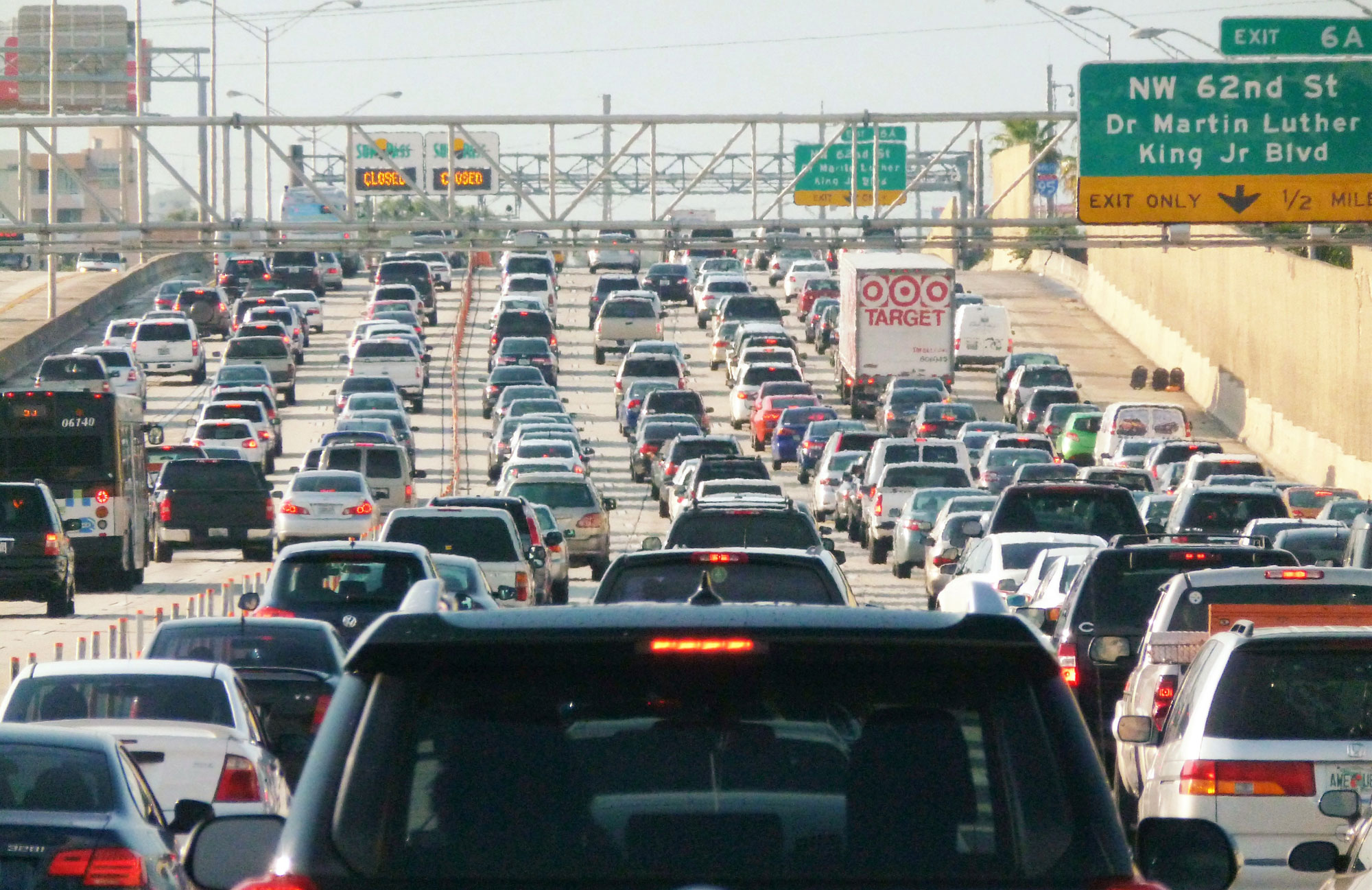
Traffic on Interstate 95-North, Miami, in 2012. Fossil fuels are being used to power the cars. Photo by B137 (Wikimedia Commons, Creative Commons Attribution-ShareAlike 4.0 International license, photo cropped and resized).
What do different units of energy mean?
Heat is energy. Measurements of heat can be thought of as the most basic way to measure energy. The British thermal unit (abbreviated BTU or BTU) is the most commonly used unit for heat energy. By definition, one BTU is approximately the amount of heat required to raise one pound of water by one degree Fahrenheit. One BTU is also about the amount of energy released by burning a single wooden match.
A joule is the energy expended (or work done) to apply a force of one newton over a distance of one meter. Since a typical apple weighs about one newton (about 100 grams or 3.6 ounces), lifting an apple one meter requires about a joule of energy. A BTU is roughly 1055 joules. That means that one BTU—the energy contained in a wooden match—is equivalent to the total amount of energy required to lift an apple 1055 meters (about 3461 feet) or a bit over one kilometer (about 0.66 or 2/3 miles).
This comparison of the energy of heat to the energy of motion—also called kinetic energy—might be a little confusing. However, energy is transformed from one type to another all the time in our energy system. This is perhaps most obvious with electricity. Electrical energy is transformed into light, heat, or motion at the flip of a switch. Those processes can also be reversed; light, heat, and motion can all be transformed into electricity. The machines that make those transformations in either direction are always imperfect, so energy always degrades into heat when it is transformed from one form to another.
Another measure of energy, the kilowatt-hour (kWh), represents the amount of energy required to light ten 100-watt light bulbs for one hour. One kWh is about 3412 BTUs or about 3.6 million joules.
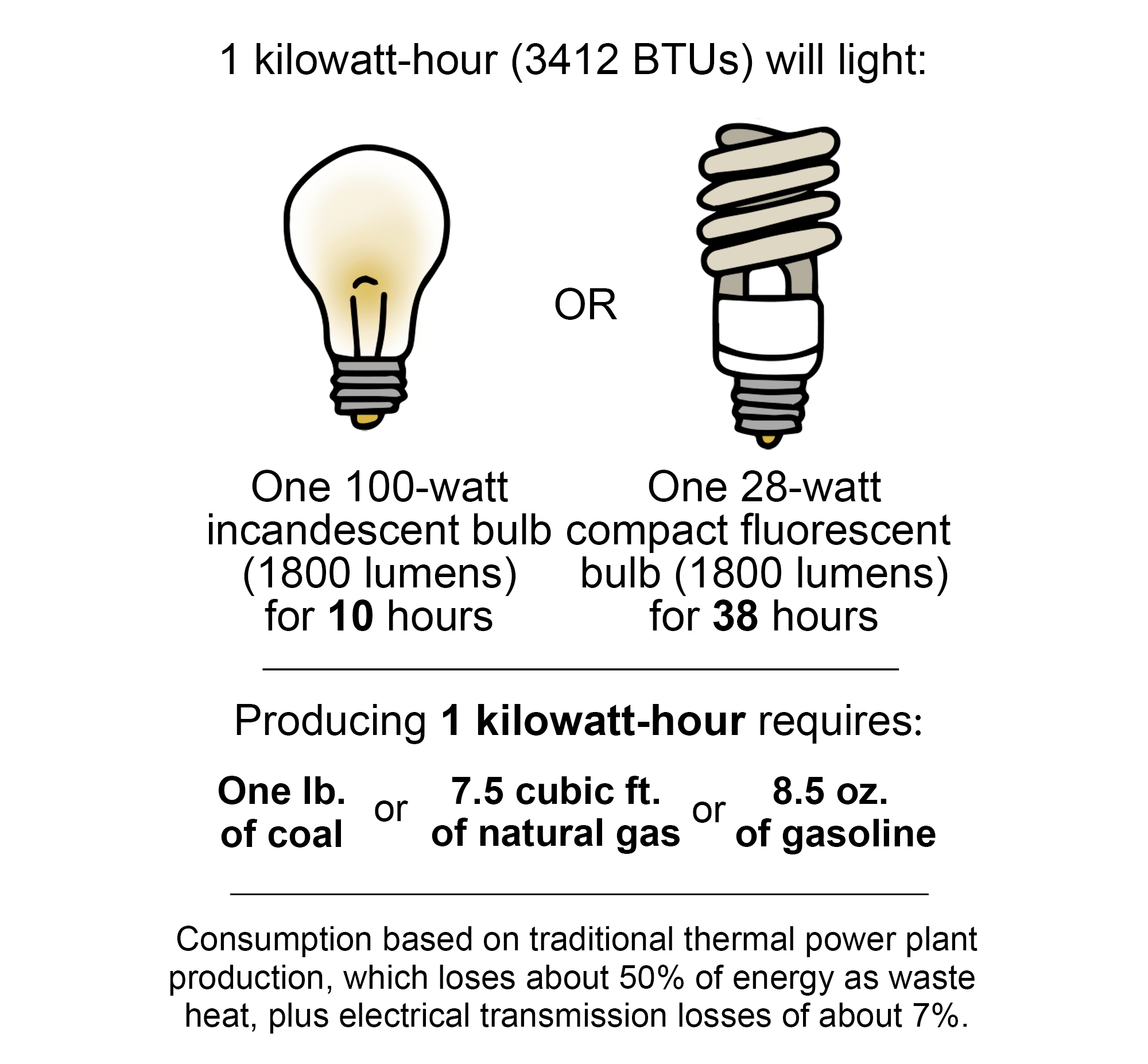
Examples of uses and sources of 1 kWh. 1kWh will light a 100-watt incandescent lighbulb for 10 hours and one 28-watt compact fluorescent bulb for 38 hours. Producing 1kWh requires one pound of coal or 7.5 cubic feet of natural gas or 8.5 ounces of gasoline. About 50% of energy used is lost as waste and 7% is lost in transmission. Image modified from original by Jim Houghton, published in The Teacher-Friendly Guide to the Geology of the Southeastern U.S., 2nd ed., edited by Andrielle N. Swaby, Mark D. Lucas, and Robert M. Ross (published by the Paleontological Research Institution) (CC BY-NC-SA 4.0 license).
How do we look at energy in the Earth system?
The Energy Information Administration (EIA) categorizes energy as coming from one of five sources: petroleum, natural gas, coal, renewable energy (for example, wind or hydroelectric), and nuclear electric power. The EIA categorizes energy as being used in one of four energy sectors: transportation, industrial, electric power, and residential and commercial). All of the energy that powers our society comes from one of these five sources and is used in one of these four sectors.
The more we come to understand the Earth system, the more we realize that there is a finite amount of consumable energy, and that harvesting certain resources for use in energy consumption may have wide ranging and permanent effects on the planet's life. Understanding energy within the Earth system is the first step to making informed decisions about energy transitions.
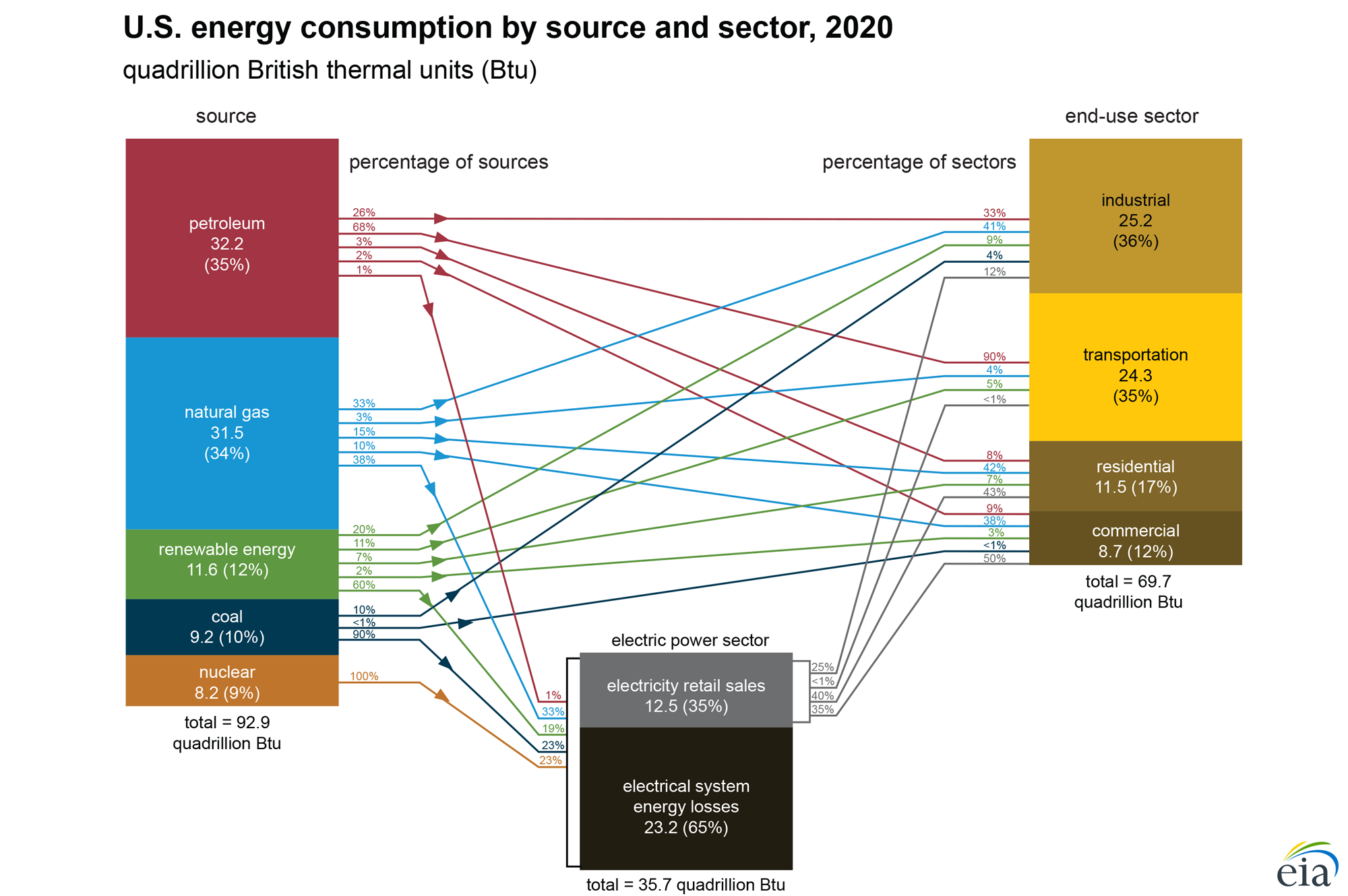
US energy production sources and use sectors for 2020. Petroleum (35%) and natural gas (34%) provide more energy than other sources. Most petroleum (68%) is used for transportation, whereas natural gas is used to produce electricity (38%) and in the industrial sector (38%). More energy is used to generate electricity than for any other use, and electricity is generated by all five major energy sources. Nuclear is unique among sources in that all of the energy it generates goes to a single sector (electricity). Image modified from"U.S. energy consumption by source and sector" by US Energy Information Administration.
Becoming "energy literate"
Energy is neither lost nor gained within the universe, but rather is constantly flowing through the Earth system. In order to fully understand energy in our daily lives and make informed decisions, we need to understand energy in the context of that system. Becoming energy literate gives us the tools to apply this understanding to solving problems and answering questions.
Energy Literacy Principles*
Each principle of energy literacy is defined by a set of fundamental concepts. Keeping these energy principles in mind when we teach others about energy can help us to place our own energy consumption in context and understand its effect on the Earth system.
- Energy is a physical quantity that follows precise natural laws.
- Physical processes on Earth are the result of energy flow through the Earth system.
- Biological processes depend on energy flow through the Earth system.
- Various sources of energy can be used to power human activities, and often this energy must be transferred from source to destination.
- Energy decisions are influenced by economic, political, environmental, and social factors.
- The amount of energy used by human society depends on many factors.
- The quality of life of individuals and societies is affected by energy choices.
*Principles from "Energy Literacy: Energy Principles and Fundamental Concepts for Energy Education," the US Office of Energy Efficiency & Renewable Energy.
Fossil fuels
Fossil fuels—oil, natural gas, and coal—are made of the preserved organic remains of ancient organisms. Organic matter is only preserved when its rate of accumulation is higher than the rate of its decay. This most often happens when the oxygen supply is so low that aerobic bacteria (oxygen-loving bacteria) cannot thrive, which greatly slows the breakdown of organic matter. When organic matter does not break down, over time it will be incorporated into buried sediment. After burial, the organic material is compacted and heated with the rest of the rock, eventually transforming it into fossil fuels.
The history of surface environments, evolution of life, and geologic processes beneath the surface have all influenced where fossil fuel deposits formed and accumulated. Coal is formed when preserved plant matter is buried, compacted, and heated. The largest coal beds were swampy environments where fallen forest trees and leaves were buried in stagnant muds. Petroleum and natural gas originate deep underground through a slow process that involves the heating of sedimentary rocks that contain an abundance of organic matter. The largest oil and gas reserves were at one time nutrient-rich seas with abundant surface phytoplankton and organic-rich bottom sediments.
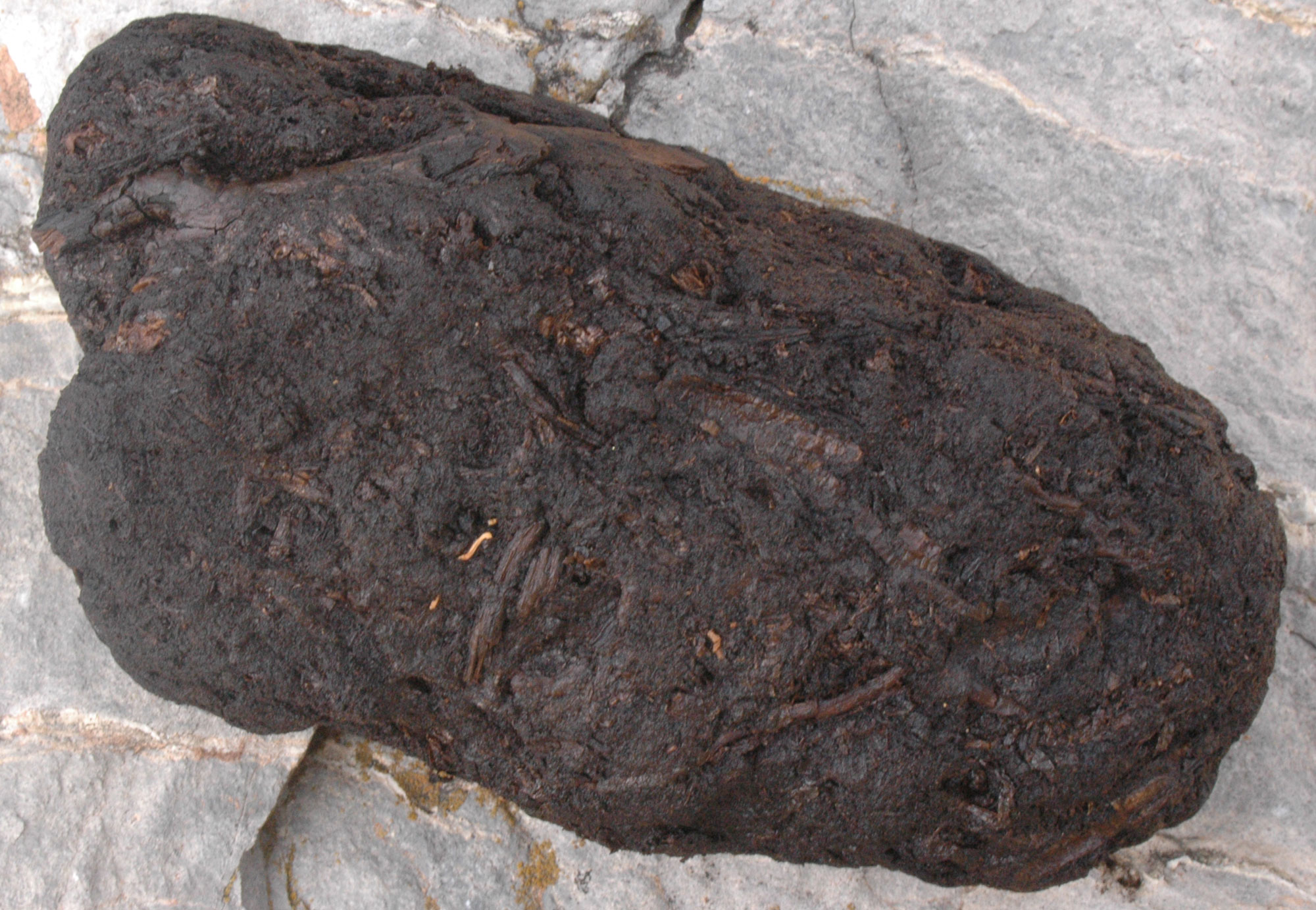
A chunk of peat. Peat is an accumulation of partially decayed plant matter. Under proper heat and pressure, it will turn into lignite coal over geologic periods of time. Photo by Donna Beaver Pizzarella, USGS (public domain).
Oil and natural gas
Oil and natural gas form from organic matter in the pores of sediments subjected to heat and pressure. The organic matter is primarily composed of photosynthetic plankton that die and sink to the bottom of large water bodies in vast numbers. Shale in particular is often organic rich, because organic matter settles and accumulates in the same places that mud (clay and silt particles) settles out of the water.
In most environments, organic matter is recycled by bacteria before it can be buried, but the quiet waters where mud accumulates are often relatively stagnant and low in oxygen. In these places, the bacterial decay rate is low relative to the rate at which organic matter sinks and becomes buried in muddy sediments. Under such conditions, organic matter may accumulate enough to make up several percent or more of the deposited sediment.
Oil and gas reservoirs
Oil and gas that form in rocks under the Earth's surface are under pressure. Therefore, they will move gradually upward to areas of lower pressure through tiny connections between pore spaces and natural fractures in rocks.
A rock layer that forms a reservoir for oil or gas must be permeable. Fluids and gas (such as water, oil, and natural gas) can move through permeable rocks, or rocks that have enough connected fractures or space between grains to form pathways for the movement of fluids and gas. Sandstone, limestone, and fractured rocks are generally permeable.
In order for a permeable rock layer to be a viable reservoir, it must also be covered by an impermeable barrier that blocks the movement of oil or gas upward out of the reservoir rock and towards the surface. Often, this barrier is formed by impermeable rock layers. Impermeable rocks are made up of tightly packed or poorly sorted particles with very little space between them. Thus, these rock layers do not have enough space for liquids and gases to travel through, and the rock layers can form a cap that traps natural gas and oil below the surface. Folds ("arches") or faults in impermeable rock layers are common barriers under which oil reservoirs form.
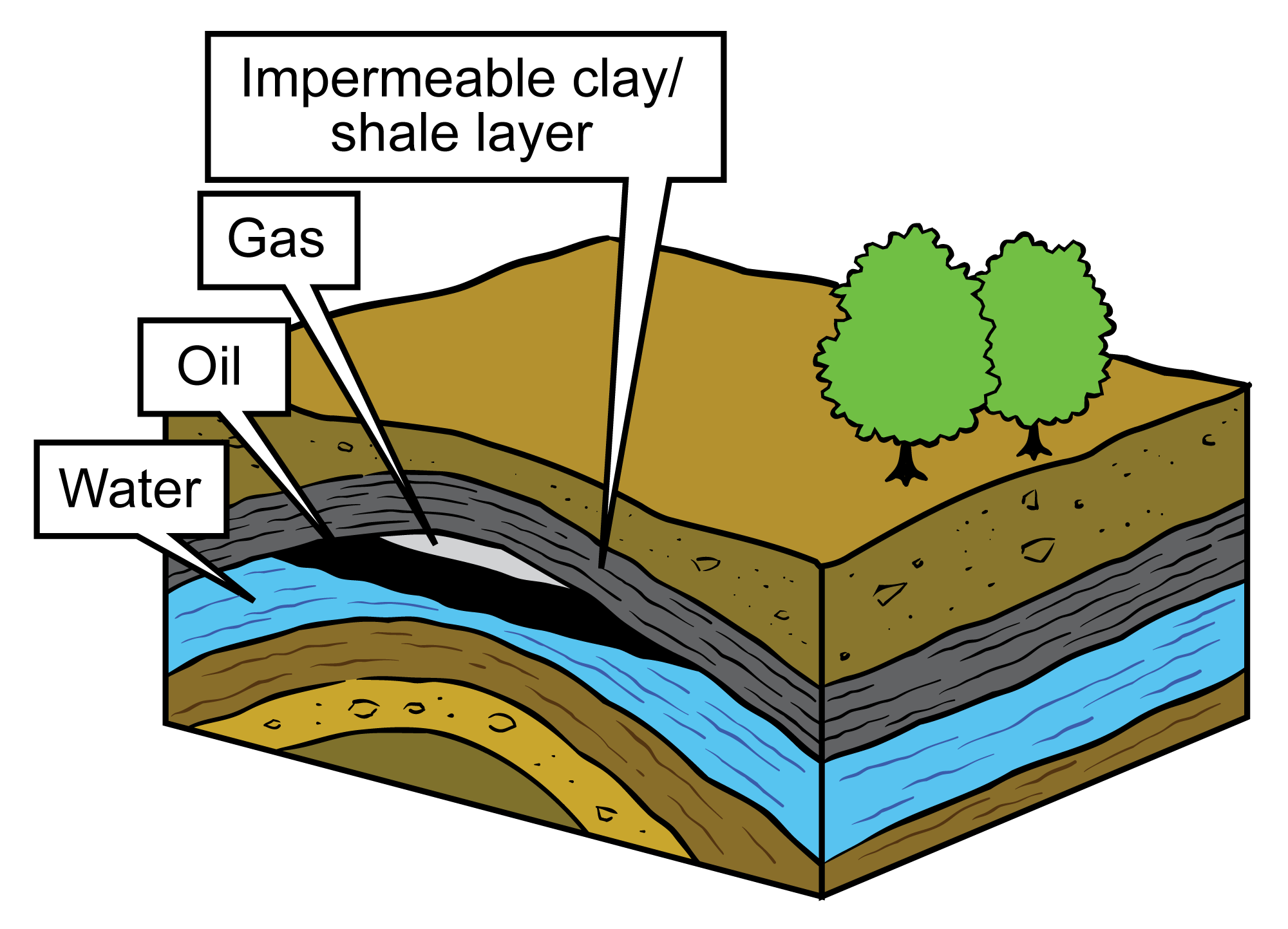
Diagram of an oil and gas reservoir. In this image, natural gas and fluids (water and oil) have accumulated in a layer of permeable reservoir rock, where they are separated by density (gas is lightest, water densest). An impermeable clay or shale layer that has been folded serves as a barrier to further movement of fluids and gas upward toward the surface. Image modified from original by Jim Houghton, published in The Teacher-Friendly Guide to the Geology of the Southeastern U.S., 2nd ed., edited by Andrielle N. Swaby, Mark D. Lucas, and Robert M. Ross (published by the Paleontological Research Institution) (CC BY-NC-SA 4.0 license).
Oil shale or shale oil?
It is unfortunate that two terms that sound as similar as "shale oil" and "oil shale" are actually quite different kinds of fossil fuel resources.
Oil shale is rock that contains an immature, waxy, solid organic material known as kerogen. Kerogen is not actually oil. Kerogen must be artificially heated to convert it into synthetic oil or a hydrocarbon gas. Thus, the whole rock layer, which may or may not technically be shale, must be mined and/or processed (possibly in place) to produce synthetic oil.
In contrast, shale oil is mature oil trapped in the original shale rock in which it formed. In this case, the source rock is also the reservoir rock, because it is so impermeable that the oil never escaped. This type of rock may be fractured (e.g., by hydraulic fracturing, discussed below) to provide pathways for the oil to escape.
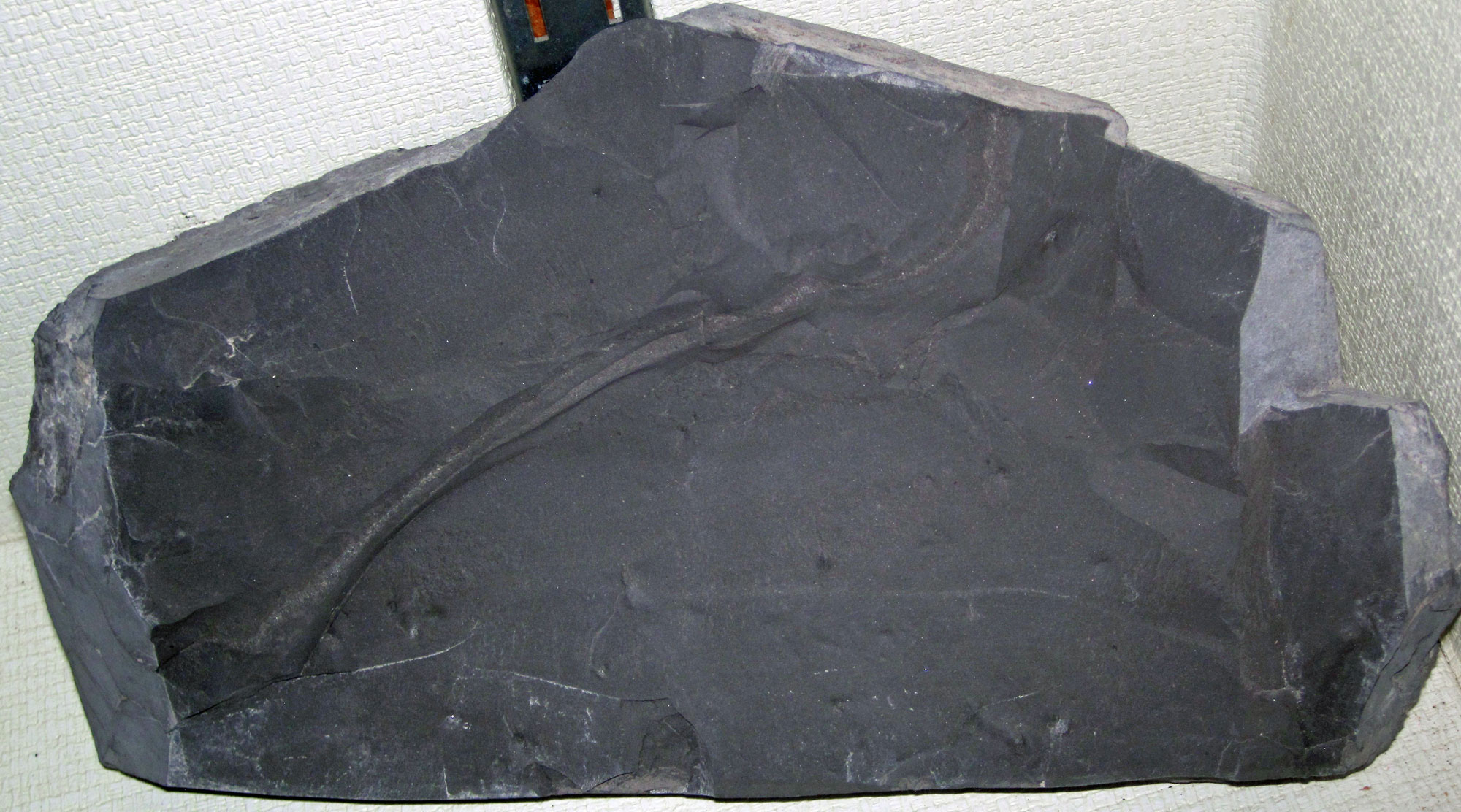
A piece of oil shale from the New Albany shale of Indiana. Photo by James St. John (flickr, Creative Commons Attribution 2.0 Generic license, image resized).
Salt domes
Rock salt (the mineral halite) is solid and impermeable, but when it is under very high pressure it can flow like a thick liquid. When a layer of salt is buried under thousands of feet of overlying sediment, it will start to deform. Because it is less dense than the rocks above it, it flows upward toward areas of lower pressure, forming geological structures named for their shapes (e.g., domes, canopies, tables, and lenses).
As salt structures grow, they in turn influence the topography of the surrounding landscape, creating zones of uplift surrounding areas of subsidence (sinking), fractures, and faults. When salt flows upward, it deforms the surrounding strata, creating gaps in which oil and gas may pool and be trapped. Oil and gas also accumulate under and along the salt structures.
Due to their inherent impermeability, the salt domes themselves are often solution-mined by pumping water underground to dissolve the salt. Solutions mining creates caverns that are used to store petroleum, gas, and even chemical waste.
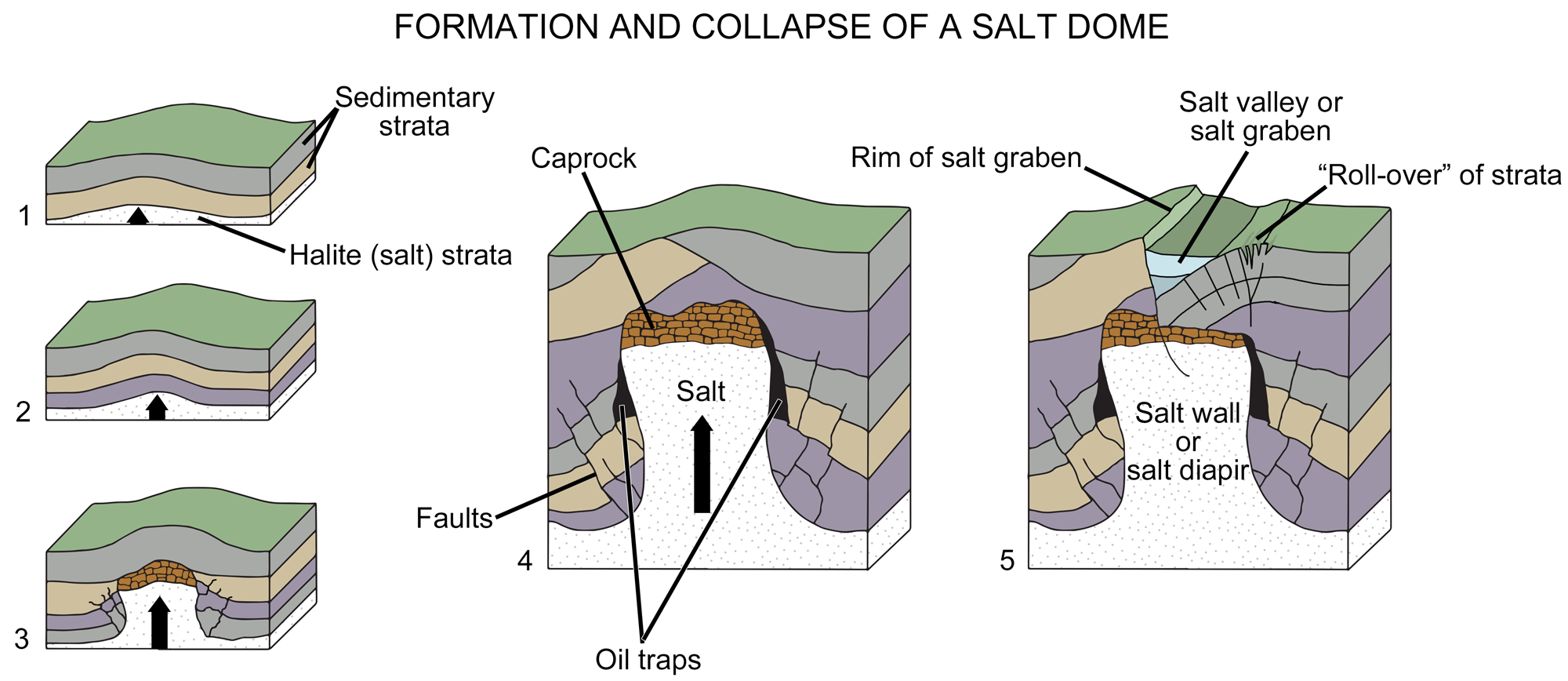
Diagram of the formation of a salt dome. In images1 to 3, a salt layer deforms, pushing upward through the rock layers above it until it is impeded by a caprock (a hard or dense rock layer). In image 4, the deformation of overlying layers by the salt has formed gaps where oil or gas has accumulated. In image 5, the rock layers under the salt have faulted and collapsed, modifying the landscape above the salt dome. Image modified from original by Wade Greenberg-Brand (after an image by Britannica online for kids), published in The Teacher-Friendly Guide to the Geology of the Southeastern U.S., 2nd ed., edited by Andrielle N. Swaby, Mark D. Lucas, and Robert M. Ross (published by the Paleontological Research Institution) (CC BY-NC-SA 4.0 license).
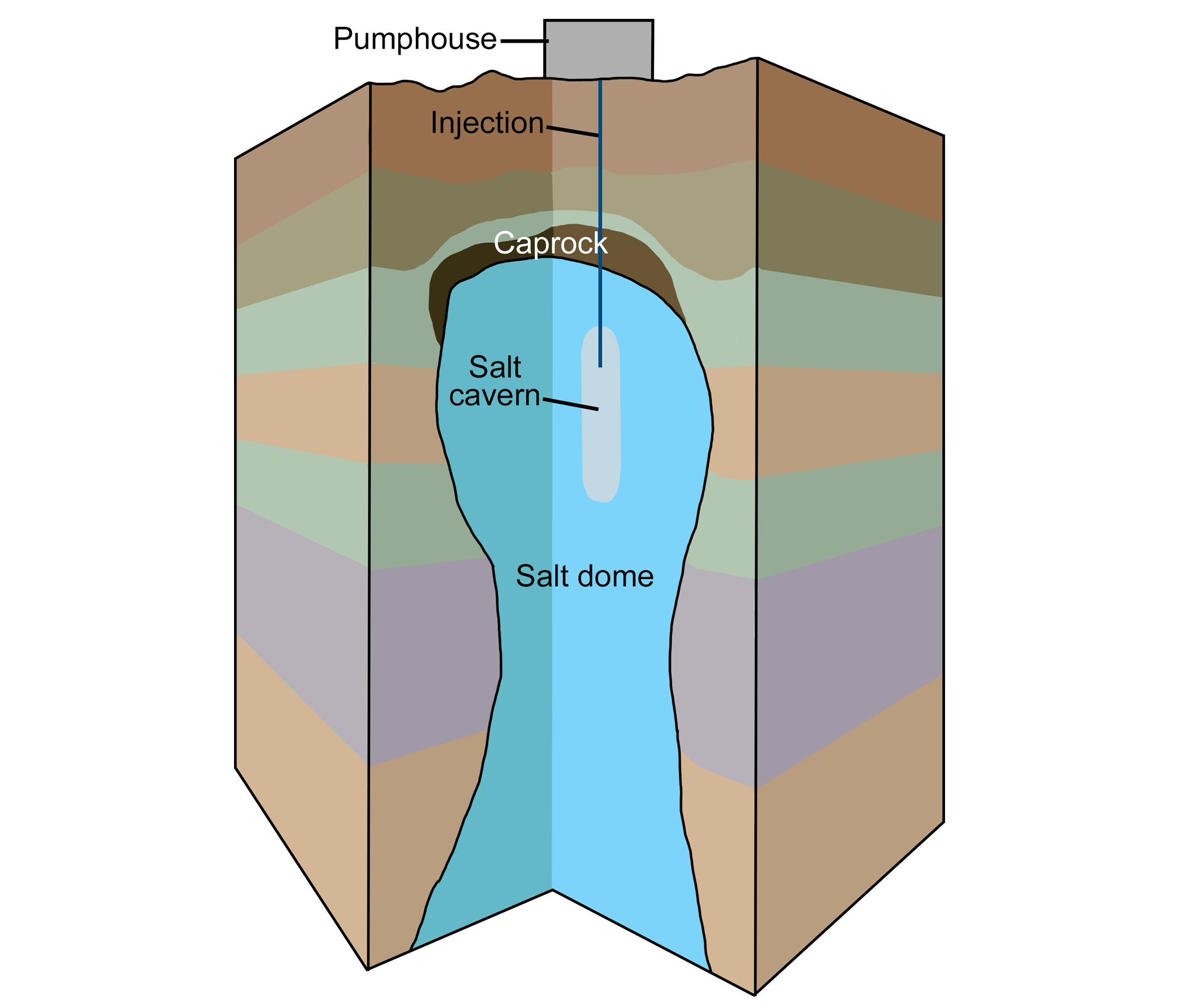
Diagram of solution mining to create a salt cavern. A pumphouse pumps water into an underground salt dome. The water dissolves the salt, and the brine (salty water) is pumped back out, creating a salt cavern (a large cavity) in the salt dome. Image modified from original by Wade Greenberg-Brand, published in The Teacher-Friendly Guide to the Geology of the Southeastern U.S., 2nd ed., edited by Andrielle N. Swaby, Mark D. Lucas, and Robert M. Ross (published by the Paleontological Research Institution) (CC BY-NC-SA 4.0 license).
Natural asphalt
Natural asphalt or bitumen deposits are oil reservoirs that have lost most of their lighter hydrocarbons, so they have become viscous, like tar. Oil that trickles out at the Earth’s surface is known as a "seep." Natural seeps of crude oil and natural gas were known to Native Americans and used in medicines before European colonization. Early European settlers used surface petroleum for medical purposes, greasing wagon wheels, softening leather, and caulking log cabins. Small local distilleries produced kerosene for lamps by the 1850s. The most famous natural asphalt seeps in the United States are the La Brea Tar Pits in Los Angeles, California.
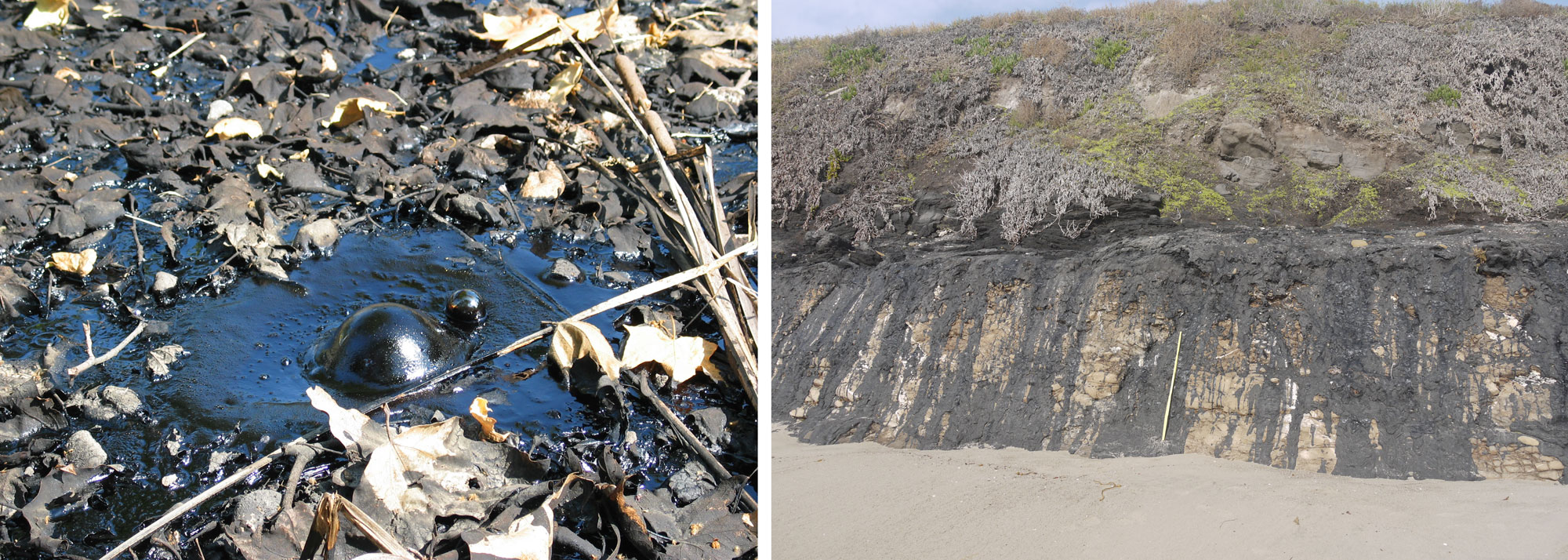
Natural asphalt seeps (tar pits) in California. Left: Bubbles in a tar pit in La Brea, Los Angeles, California. Photo by Daniel Schwen (Wikimedia Commons, Creative Commons Attribution license 2.5 Generic license, image cropped). Right: Asphalt seep in Carpinteria, Califronia. Photo by Ipab (Wikimedia Commons, Creative Commons Attribution-Share Alike 4.0 International license, image cropped and resized).
Oil drilling
Conventional wells
Once an oil trap or reservoir rock has been detected on land, oil crews excavate a broad, flat pit for equipment and supplies around the area where the well will be drilled. Once the initial hole is prepared, an apparatus called a drilling rig is set up. The rig is a complex piece of machinery designed to drill through rock to a predetermined depth. A typical drilling rig usually contains generators to power the system, motors and hoists to lift the rotary drill, and circulation systems to remove rock from the borehole and lubricate the drill bit with mud.
Gushers were an icon of oil exploration during the late 19th and early 20th centuries. These occurred when highly pressurized reservoirs were breached by simple drilling techniques. Oil or gas would travel up the borehole at a tremendous speed, pushing the drill bit out and spewing out into the air. Although iconic, gushers were extremely dangerous and wasteful. As well as spewing thousands of barrels of oil onto the landscape, they were responsible for the destruction of life and equipment. The advent of specialized blowout prevention valves in the 1920s enabled workers to prevent gushers and to regain control of blown wells. Today, this equipment is standard in both on- and offshore oil drilling.
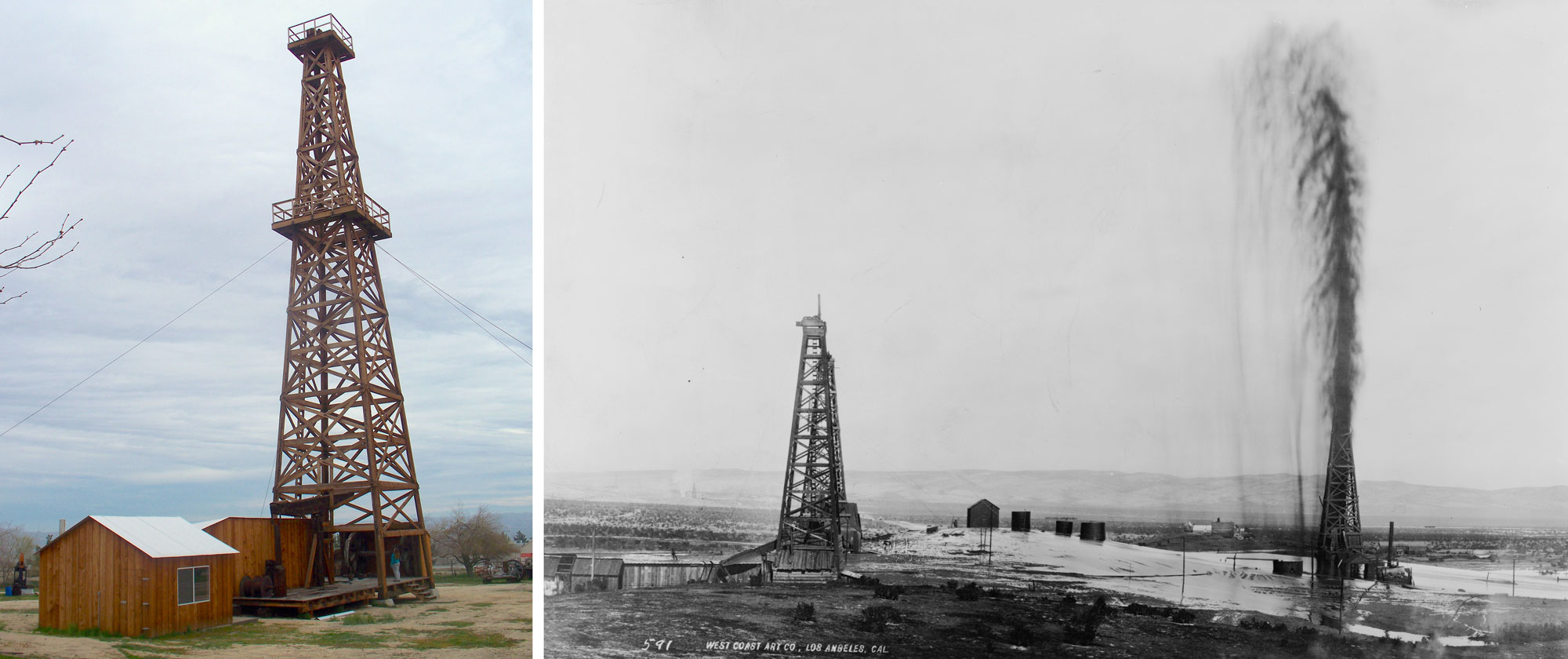
Historical oil derricks (derricks are the tower-like structures). Left: Wooden derrick built ca. 1917 preserved at the West Kern Oil Museum in California. Photo by Konrad Summers (Wikimedia Commons, Creative Commons Attribution-Share Alike 2.0 Generic license, image resized and cropped). Right: An oil field in California ca. 1910 with a gusher spewing oil on the right. Photo by West Coast Art Co. (from the Library of Congress Prints and Photographs Online Catalog, no known restrictions on publication).
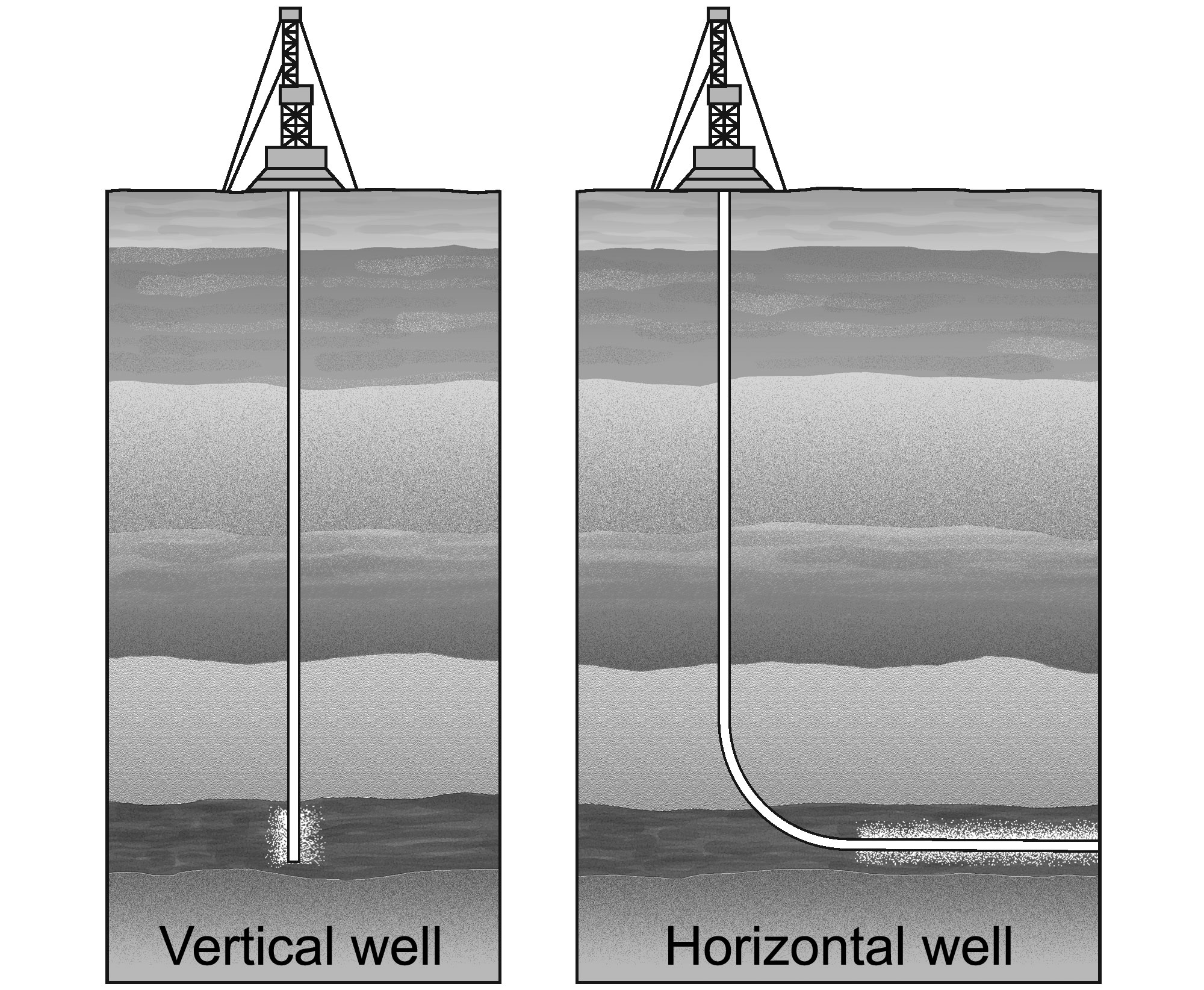
Diagrams of oil wells. Left: A conventional vertical well. Right: A horizontal well. Hydraulic fracturing may be carried out along horizontal wells running for 1.6 kilometers (1 mile) or more along layers with oil or gas trapped in pore spaces. Image modified from original by Jim Houghton, published in The Teacher-Friendly Guide to the Geology of the Southeastern U.S., 2nd ed., edited by Andrielle N. Swaby, Mark D. Lucas, and Robert M. Ross (published by the Paleontological Research Institution) (CC BY-NC-SA 4.0 license).
The support structure used to hold the drilling apparatus is called a derrick. In the early days of oil exploration, drilling rigs were semi-permanent structures and derricks were left onsite after the wells were completed. Today, however, most rigs are mobile and can be moved from well to well. Once the well has been drilled to a depth just above the oil reservoir, a cement casing is poured into the well to structurally reinforce it. Once the casing is set and sealed, oil is then allowed to flow into the well, the rig is removed, and production equipment can be put in place to extract the oil.
Offshore drilling follows much the same process as onshore drilling but utilizes a mobile offshore drilling unit (MODU) to dig the well. There are several different types of MODUs, including submersible units that sit on the sea floor, drilling ships, and specialized rigs that operate from atop floating barges.
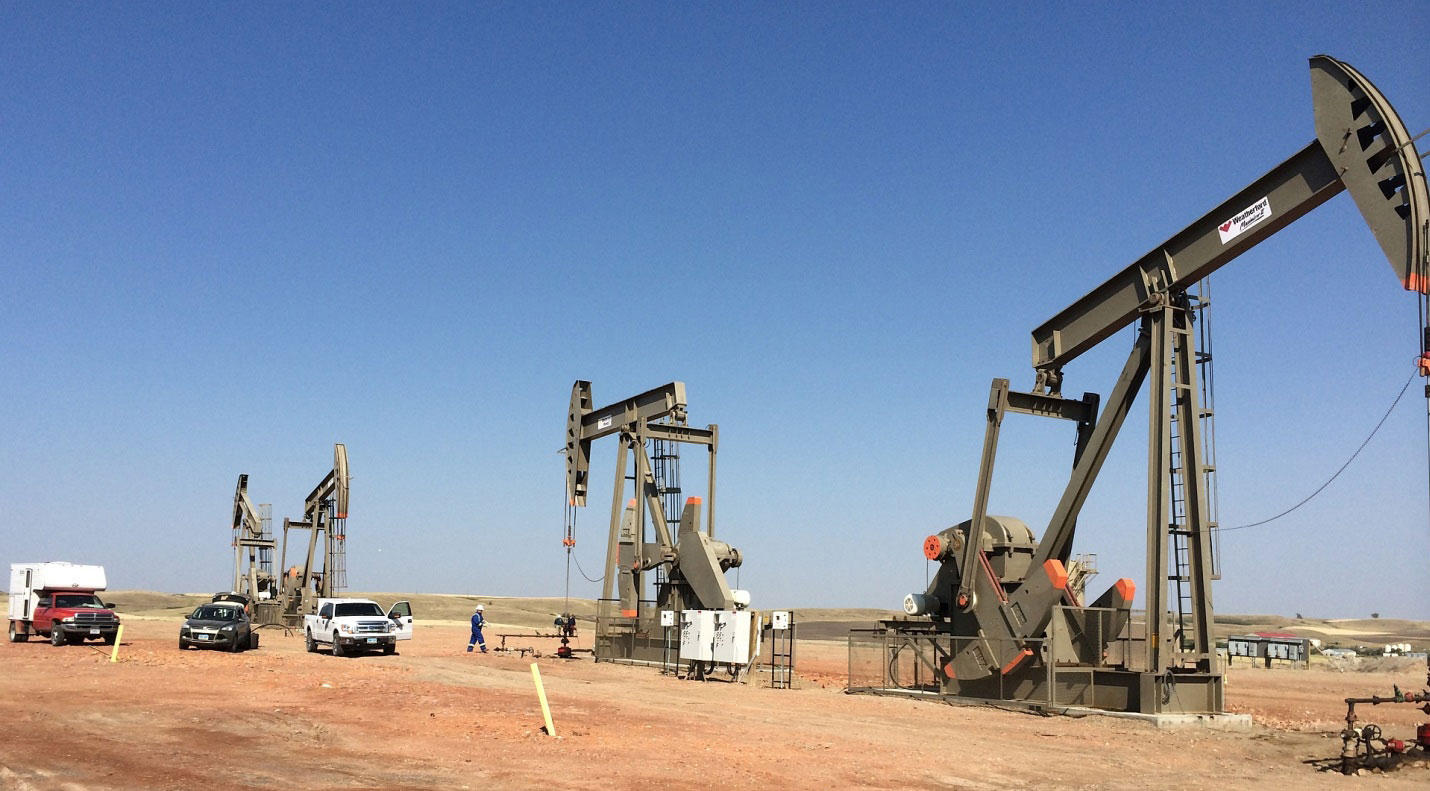
Pumpjacks at oil wells in the Bakken Formation, North Dakota. In these modern oil wells, the derricks used to drill the wells have been removed. Photo by USGS (public domain).
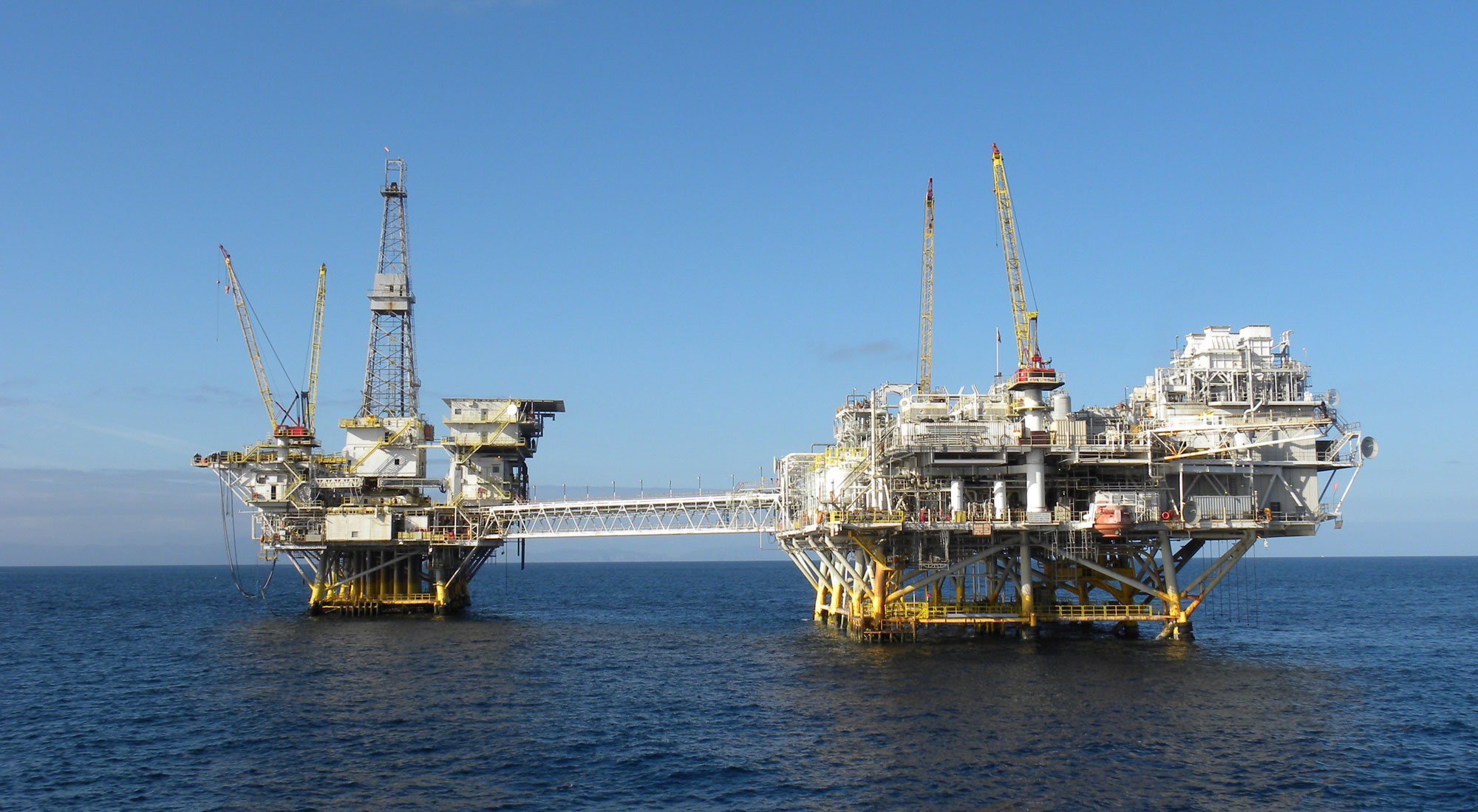
Oil platforms off the coast of California. Photo by Bureau of Safety and Environmental Enforcement (flickr, public domain).
Hydraulic fracturing (“fracking”)
Devonian-aged shales are the major source rock for petroleum and natural gas. Because the shales are not permeable, gas production occurs where the rocks are naturally fractured or where rocks are intentionally fractured using a process called hydraulic fracturing. When source rocks with low permeability—also known as "tight" layers—are fractured beneath the surface, gas and oil trapped within them are released.
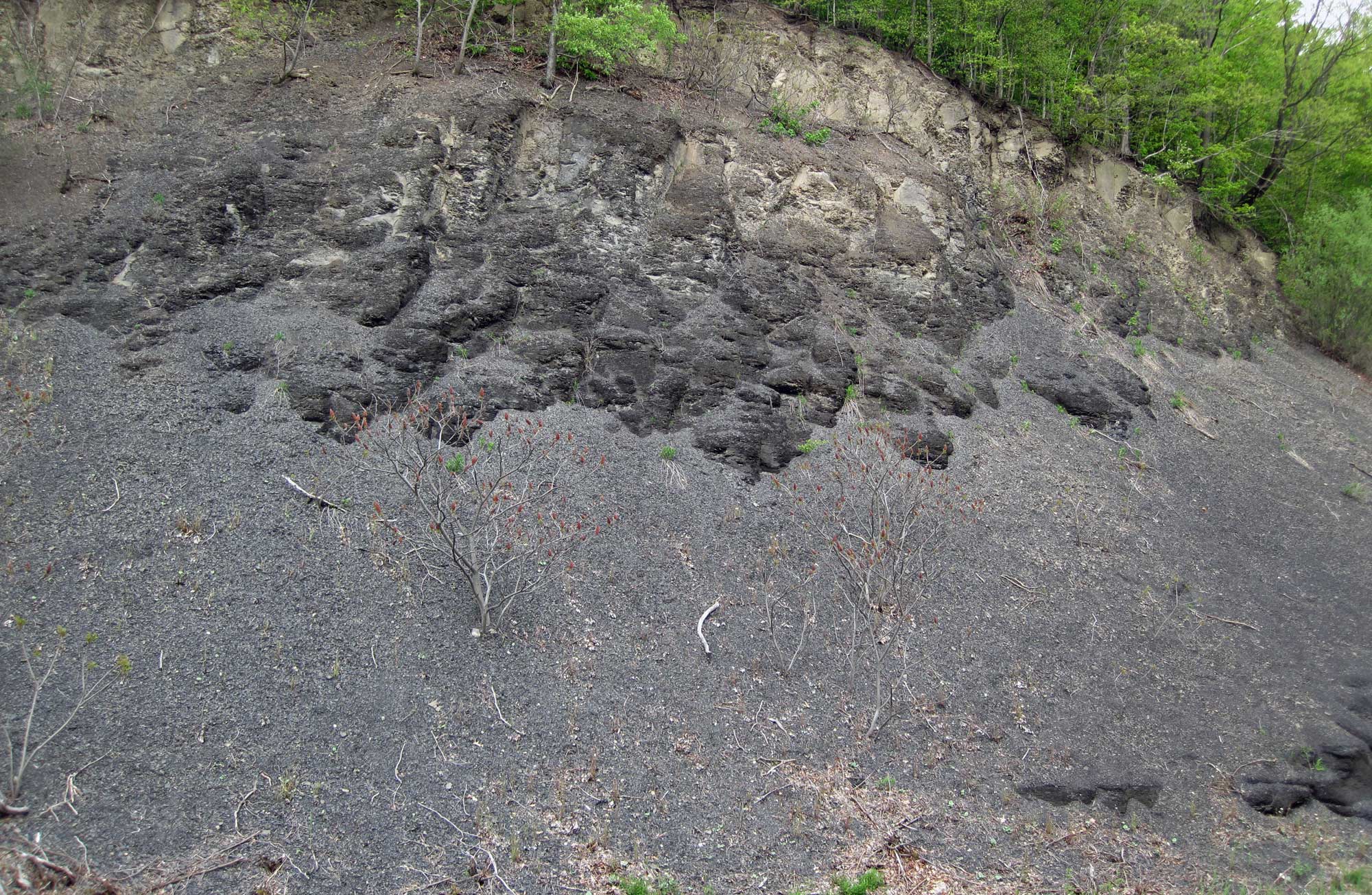
Devonian Solsville Shale Member, Marcellus Shale Formation, New York. The Marcellus Shale is a gas-producing shale that occurs primarily in New York, Ohio, Pennsylvania, and West Virginia. It is exploited for gas in some regions using hydraulic fracturing. Photo by James St. John (flickr, Creative Commons Attribution 2.0 Generic license, image cropped and resized).
Hydraulic fracturing uses horizontal wells drilled along the source rock layer. Most horizontal wells are drilled where the source rock is about 100–150 meters (330–490 feet) thick. The source rocks are fractured using high volumes of fracking fluid (frac fluid) flushed through the well at high pressure. The frac fluid is made up of water mixed with gel, sand, and chemicals. The gel increases the viscosity of the fluid. The thousands of tiny fractures created by the fluid are held open by the small grains of sand.
Chemicals are added to the frac fluid to increase the recovery of fossil fuels. One type of chemical is "slickwater," which is used to reduce friction. "Slickwater, high-volume hydraulic fracturing"—often shortened to "hydraulic fracturing" or simply "fracking"—has greatly increased the accessibility of fossil fuel resources and the production rate of oil and gas.
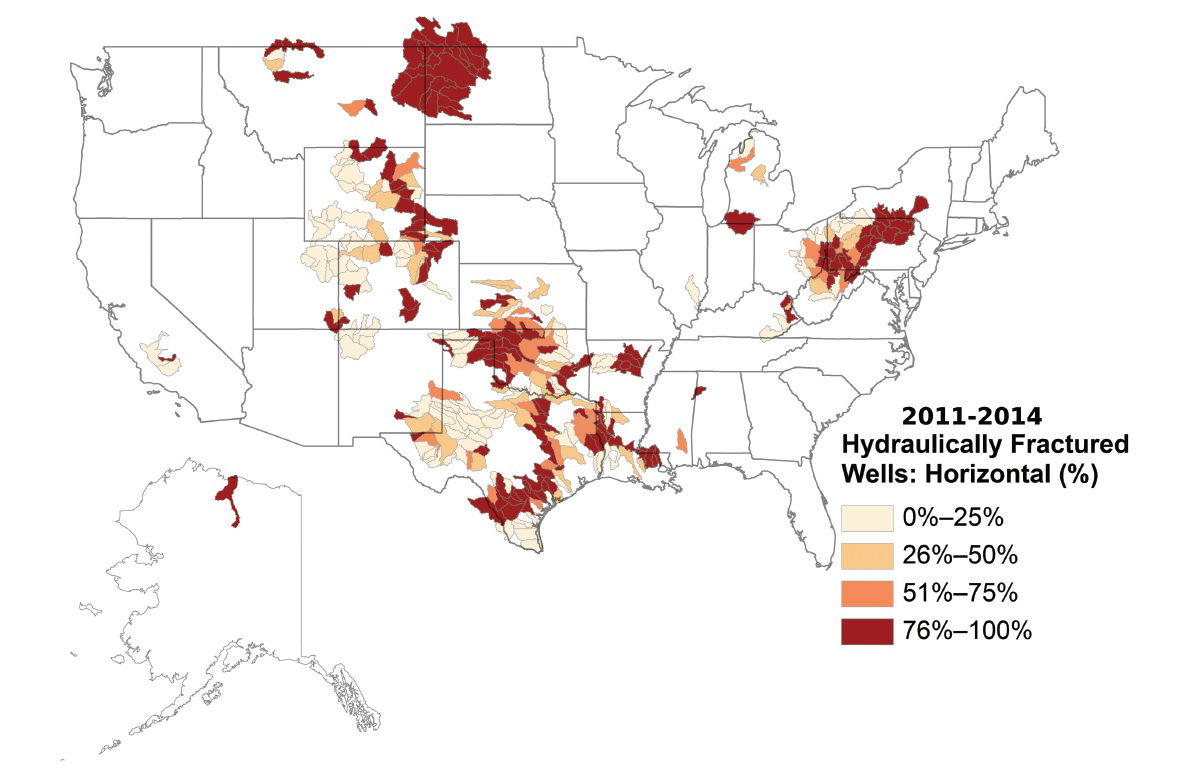
Percentage of hydraulically fractured (horizontally drilled) wells in oil and gas producing areas of the United Sates. Map by the USGS.
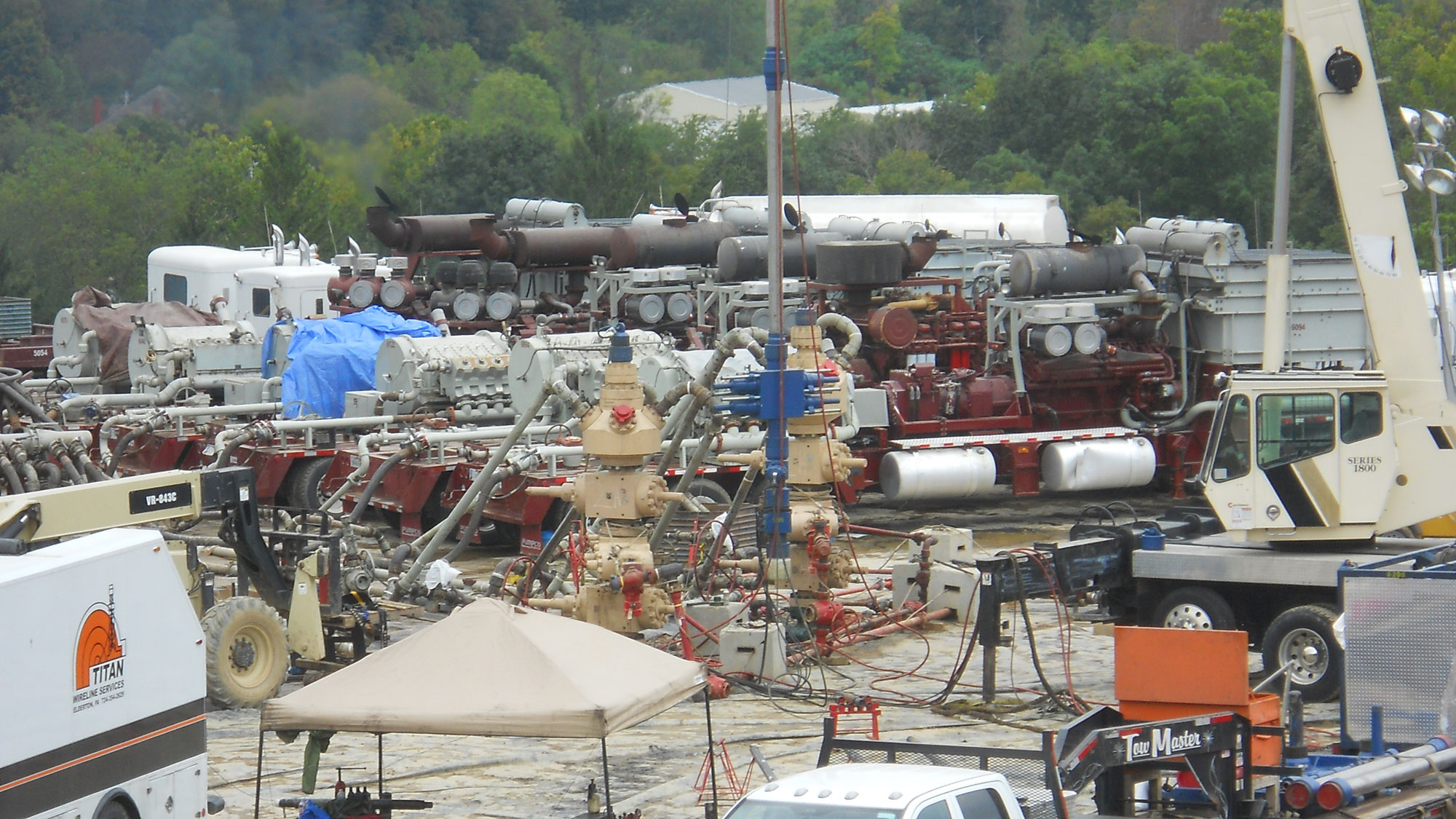
Hydraulic fracturing in progress in the Marcellus Shale of Pennsylvania. The Marcellus Shale is a Devonian-aged, gas-producing shale that occurs primarily in New York, Ohio, Pennsylvania, and West Virginia. Photo by Doug Duncan, USGS (public domain).
Fracking has been controversial, in large part because of associated impacts. For example, fracking and other oil and gas extraction activities create large quantities of wastewater that contain salts and other contaminants. This wastewater may include frac fluid and produced water. Produced water is water that is pumped out of the ground along with oil or gas. This contaminated water must be treated, reused, or contained.
One method of wastewater containment is the use of injection wells. Injection wells are used to pump wastewater deep underground. Underground disposal of wastewater using injection wells has caused powerful earthquakes in some areas of the US that have experienced few powerful earthquakes before. Induced earthquakes (earthquakes caused by human activity) are especially a problem where buildings and other infrastructure have not been built to withstand shaking.
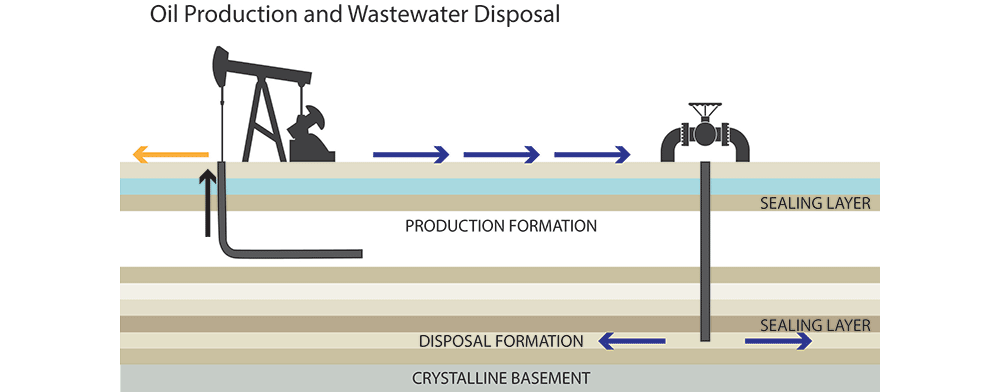
Diagram showing pumping of oil (left) and injection of wastewater into a well (right). Note that wastewater is injected deep underground beneath a sealing layer of impermeable rock. Source: USGS (public domain).
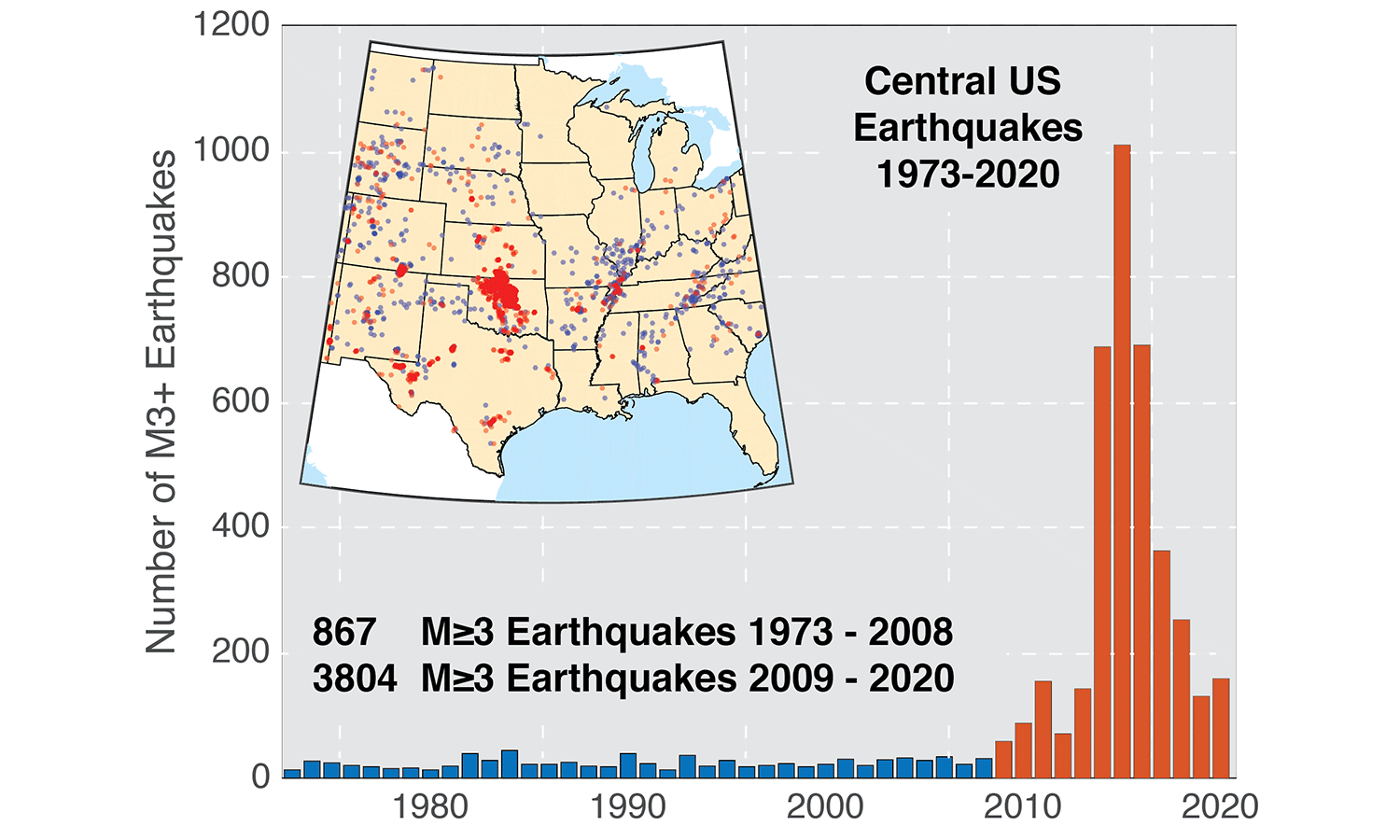
Original description from the USGS: "Annual number of earthquakes with a magnitude of 3.0 or larger in the central and eastern United States, 1973–2020. The long-term rate of approximately 25 earthquakes per year increased sharply starting around 2009." Source: USGS (public domain).
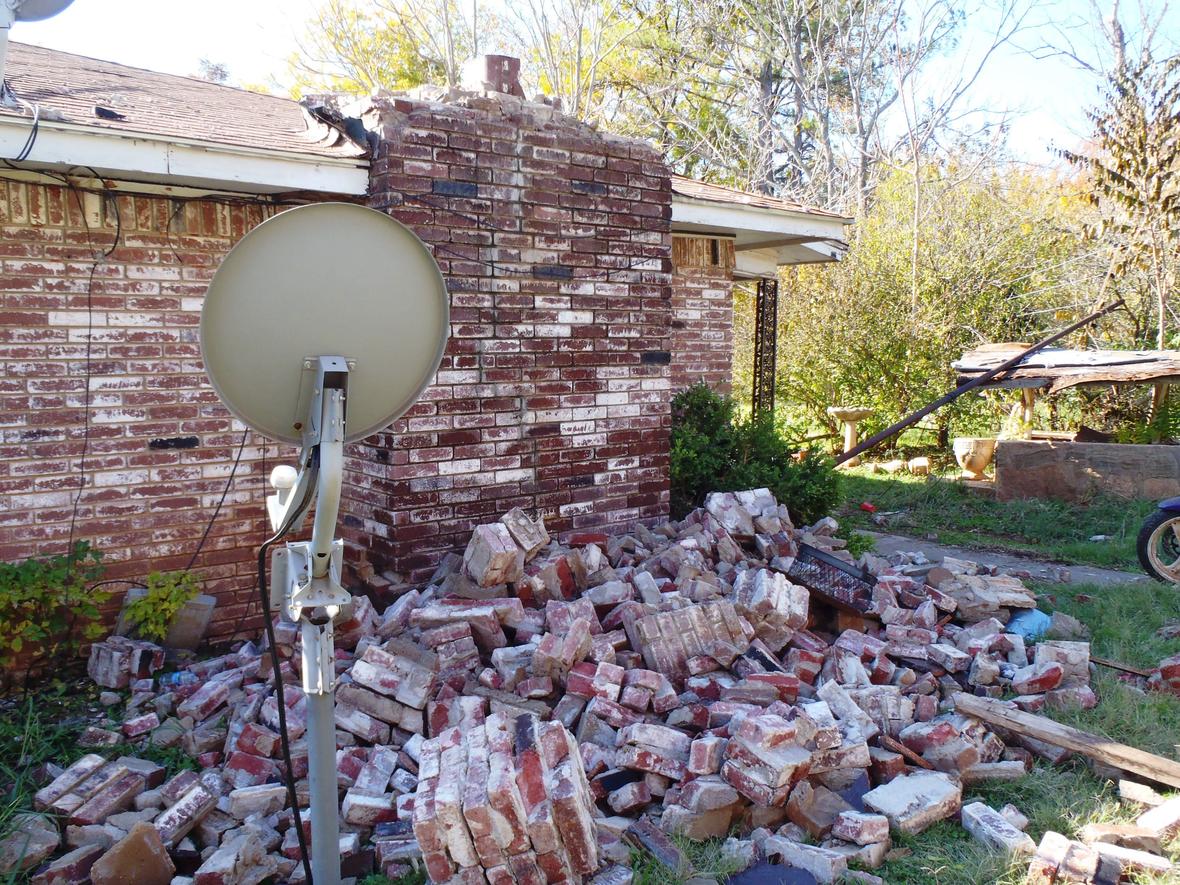
Original description from the USGS: "House damage in central Oklahoma from the magnitude 5.6 earthquake on Nov. 6, 2011. Research conducted by USGS geophysicist Elizabeth Cochran and her university-based colleagues suggests that this earthquake was induced by injection into deep disposal wells in the Wilzetta North field." Photo by Brian Sherrod, USGS.
Coal
How does coal form?
Coal ultimately comes from organic matter from land plants. Leaves, wood, and other plant matter accumulate on the ground as plants die or shed parts. If these structures do not rapidly decay, they may form peat, an accumulation of partially decayed plant matter. The peat may then be buried by additional organic matter and sediment. As the peat is buried more and more deeply by additional layers of sediment and organic matter, pressure from the overlying sediments builds, squeezing and compressing the peat into coal.
Over time, the coal may become more carbon rich as water and other components are squeezed out. Peat may become lignite, bituminous, and eventually anthracite coal. By the time a peat bed has been turned into a layer of anthracite, they layer is one-tenth of its original thickness and is up to 95% carbon. Anthracite has the fewest pollutants of the four types of coal, because it has the highest amount of pure carbon.
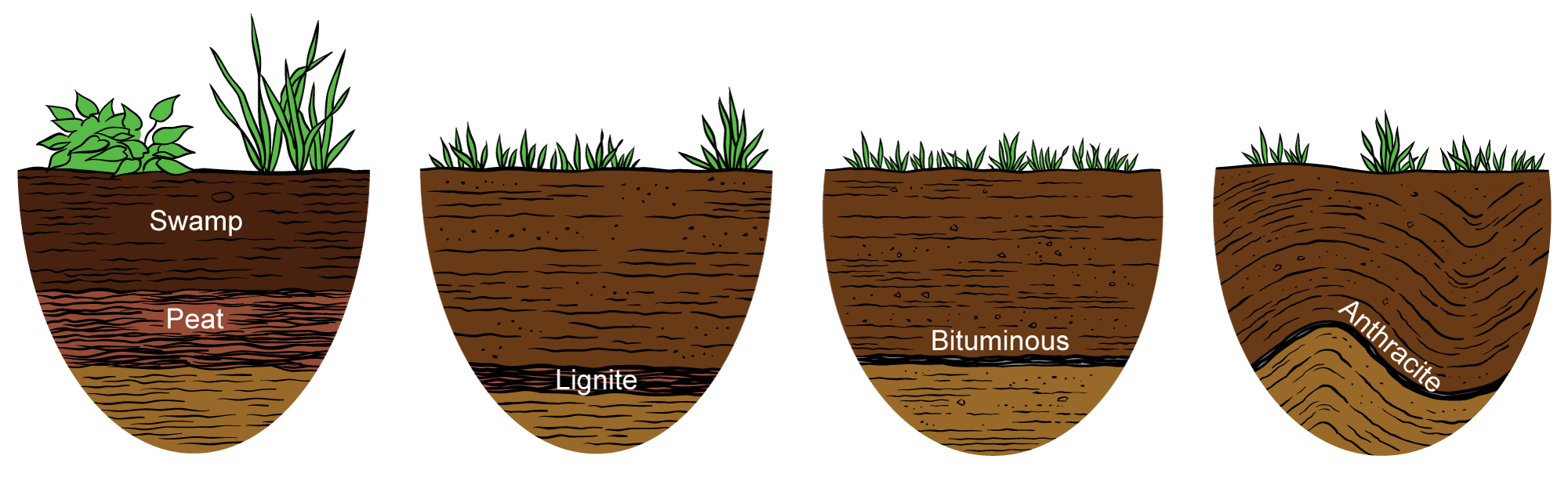
Stages in the formation of coal, from left to right: Peat is buried beneath a swamp. Through compaction and loss of water, it forms lignite. Through further compression, the coal transforms into bituminous coal and finally anthracite coal. Image modified from original by Jim Houghton, published in The Teacher-Friendly Guide to the Geology of the Southeastern U.S., 2nd ed., edited by Andrielle N. Swaby, Mark D. Lucas, and Robert M. Ross (published by the Paleontological Research Institution) (CC BY-NC-SA 4.0 license).
When did coal from?
The Carboniferous period takes its name from the carbon in coal. Globally, a remarkable amount of today's coal formed from the plants of the Carboniferous. These plant formed thick forests ("coal swamps") that were dominated by large trees. Coals deposited during the Pennsylvanian period occur in repeated successions of sedimentary rock layers known as cyclothems, which are alternating sequences of marine and non-marine sedimentary rocks. Carboniferous cyclothems formed due to repeated sea level changes caused by the growth and melting of continental glaciers on the supercontinent Gondwana from about 330 to 260 million years ago.
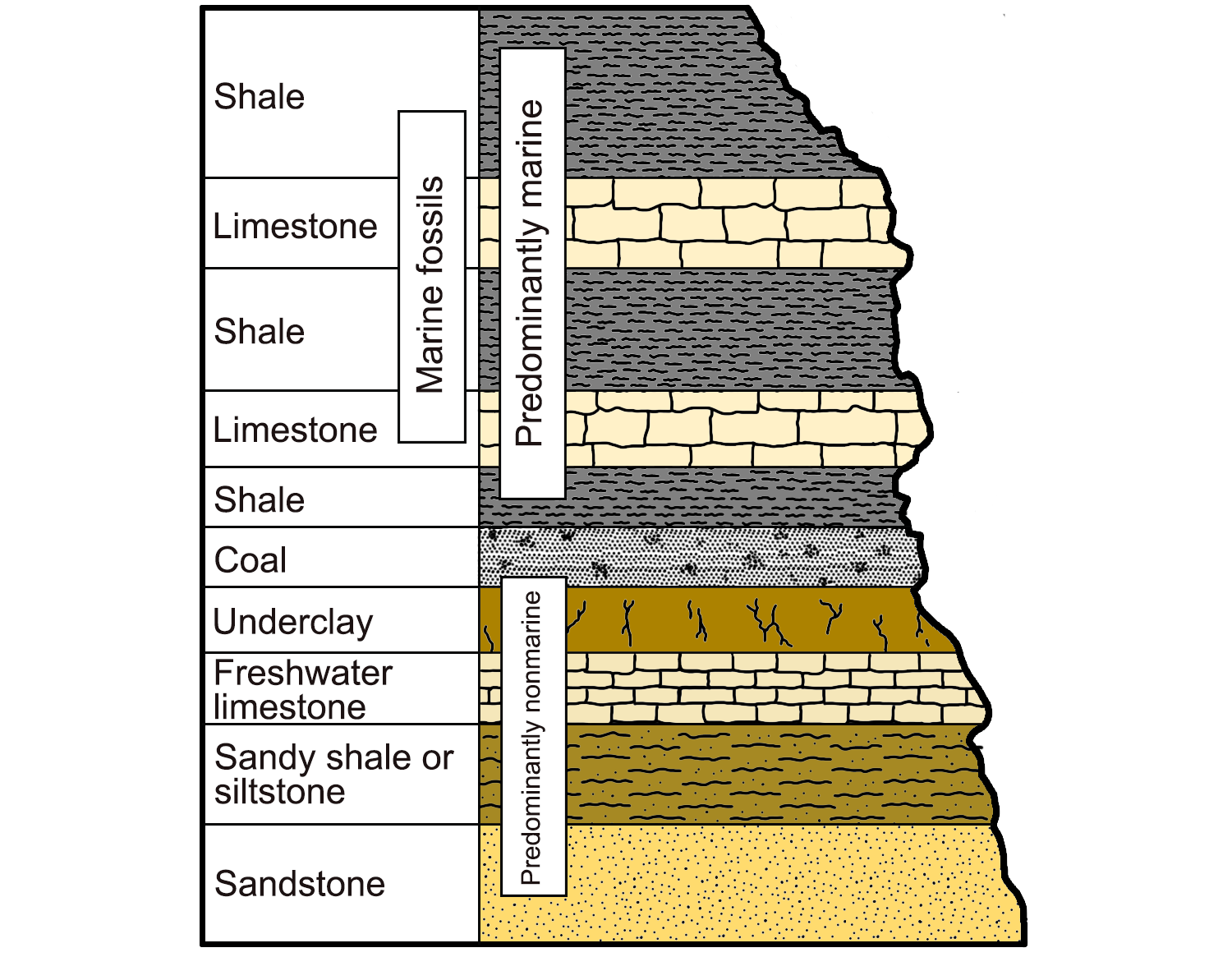
An example of a cyclothem, alternating sequences of marine and nonmarine sedimentary rocks characterized by their light and dark colors. Image modified from original by Wade Greenberg-Brand (after image from Levin, 2006, The Earth Through Time, 8th ed.), published in The Teacher-Friendly Guide to the Geology of the Southeastern U.S., 2nd ed., edited by Andrielle N. Swaby, Mark D. Lucas, and Robert M. Ross (published by the Paleontological Research Institution) (CC BY-NC-SA 4.0 license).
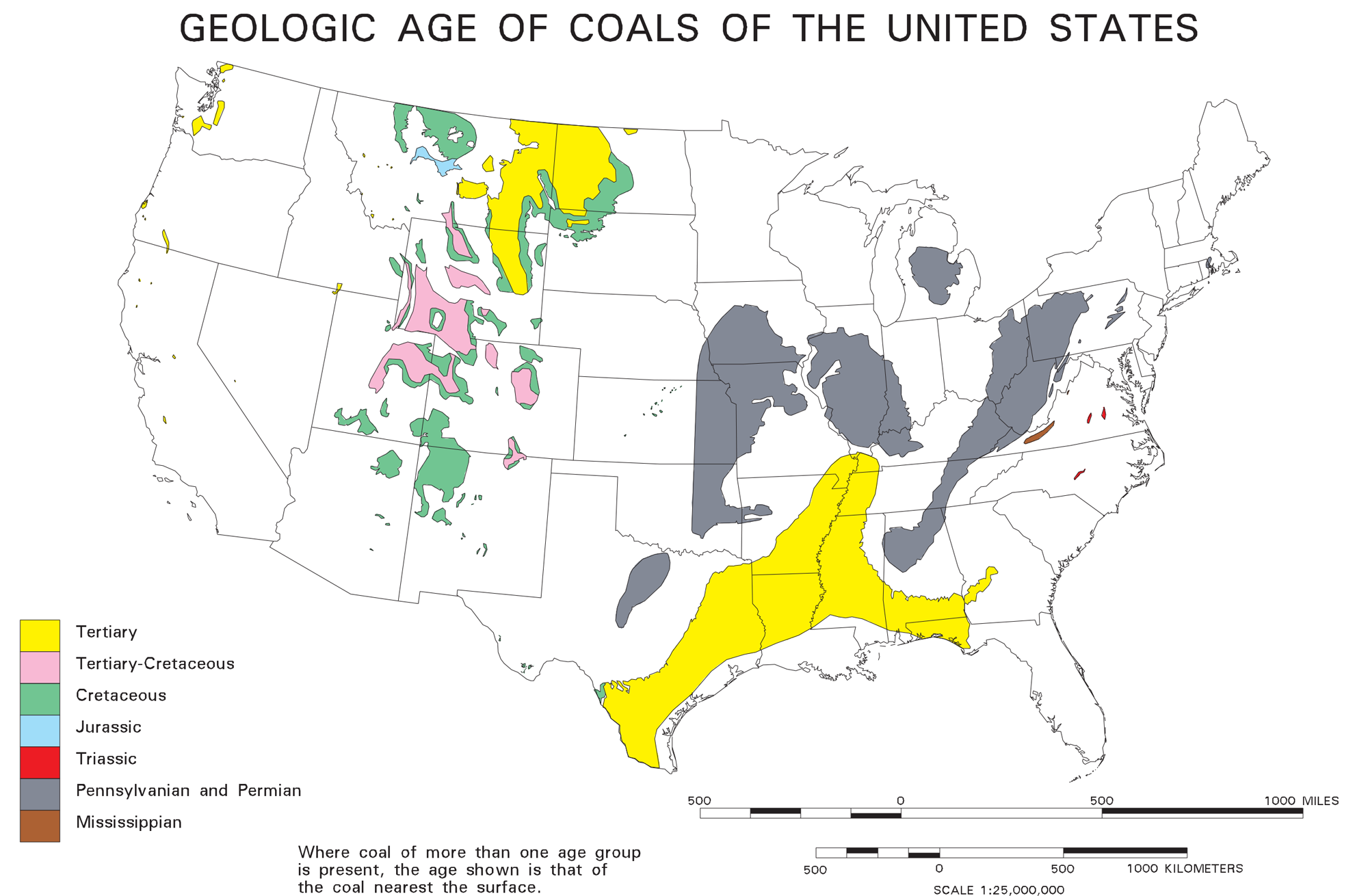
Coal deposits and the ages of coals in the contiguous US. The Tertiary period is the former name for the time interval now split into the Paleogene and Neogene periods. Source: USGS Open-File Report 96-92, digital compilation by John Tully.
Thick coal deposits are not found in coastal deposits and deltas that formed during earlier times even though geologic and climatic conditions were similar. This is because the plants that made up the coastal swamp forests that produced enough biomass to form large peat deposits had not yet evolved. Plants had only just begun to spread on to land and evolve vascular tissue during the Silurian period. Diversification and evolution of plants during the Devonian was rapid. As forests evolved and increased in size in the late Devonian and Carboniferous, significant quantities of organic matter were produced on land for the first time. The Carboniferous is not the only time period during which large coal deposits formed. Coals are also known from the Mesozoic and Cenozoic eras.
During the Carboniferous, burial of enormous quantities of terrestrial organic matter took carbon dioxide (CO2) out of the atmosphere. CO2 concentrations decreased to the point that global cooling led to the growth of continental glaciers. Today, we are enacting the same process in reverse. In only a few hundred years, we have released carbon dioxide that took millions of years to be buried into the atmosphere.
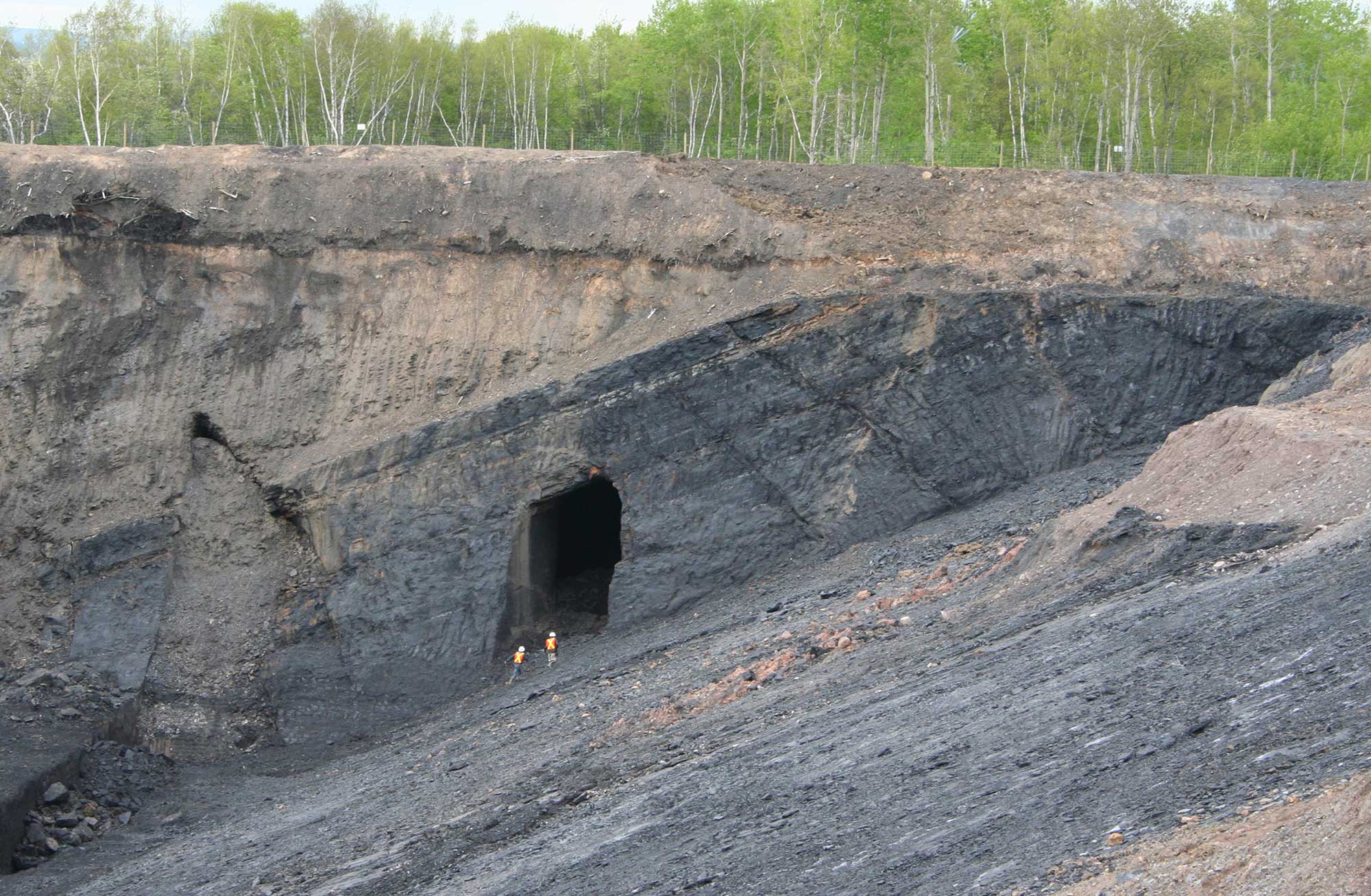
Foord Coal Seam, Stellarton Basin, Nova Scotia, Canada. This Pennsylvanian (late Carboniferous) seam of bituminous coal is 11 to 13 meters thick (about 36 to 43 feet thick). Photo by Michael C. Rygel (Wikimedia Commons, Creative Commons Attribution Share-Alike 3.0 Unported license, image cropped and resized).
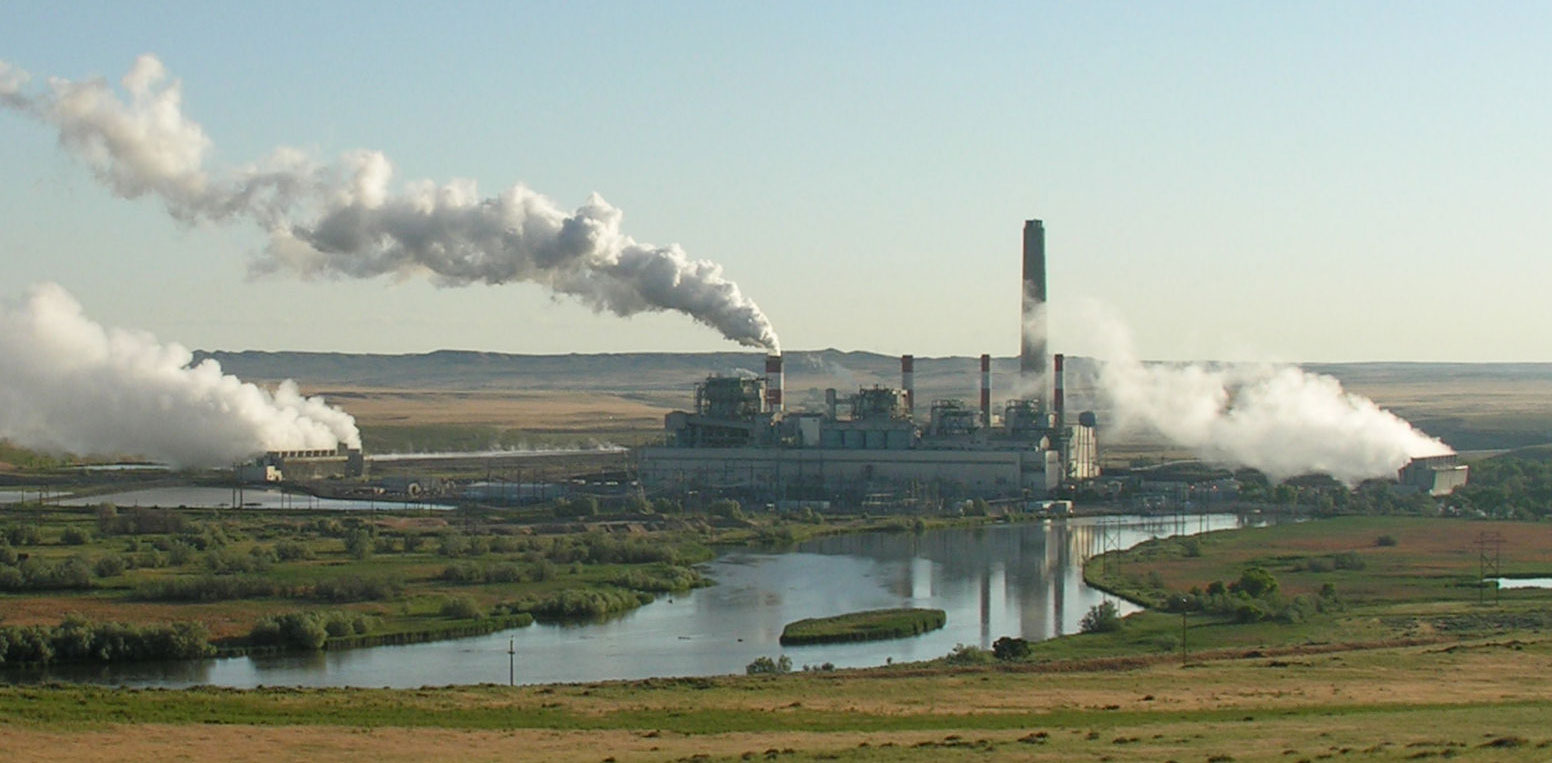
Dave Johnston Power Plant, a coal-fired power plant in Wyoming, photographed in 2006. Photo by Greg Goebel (flickr, Creative Commons Attribute-ShareAlike 2.0 Generic).
Coalbed methane
Since about 1980, large reserves of natural gas have been exploited in tandem with coal seams. This gas, called coalbed methane, is a byproduct of the process of coalification (coal formation). During coal mining, coal seams (deposits) have long been vented, in part because of the potential build-up of methane (CH4, the primary gas in "natural gas") released from fissures around the coal. Methane is a safety hazard in subsurface mines. The build-up of methane in mine shafts can cause explosions if the gas is ignited; an ignition source could be a spark, for example. Coal mine explosions have occurred throughout the history of coal mining in the US and have killed thousands of miners. (Another cause of coal mine explosions is ignition of coal dust.)
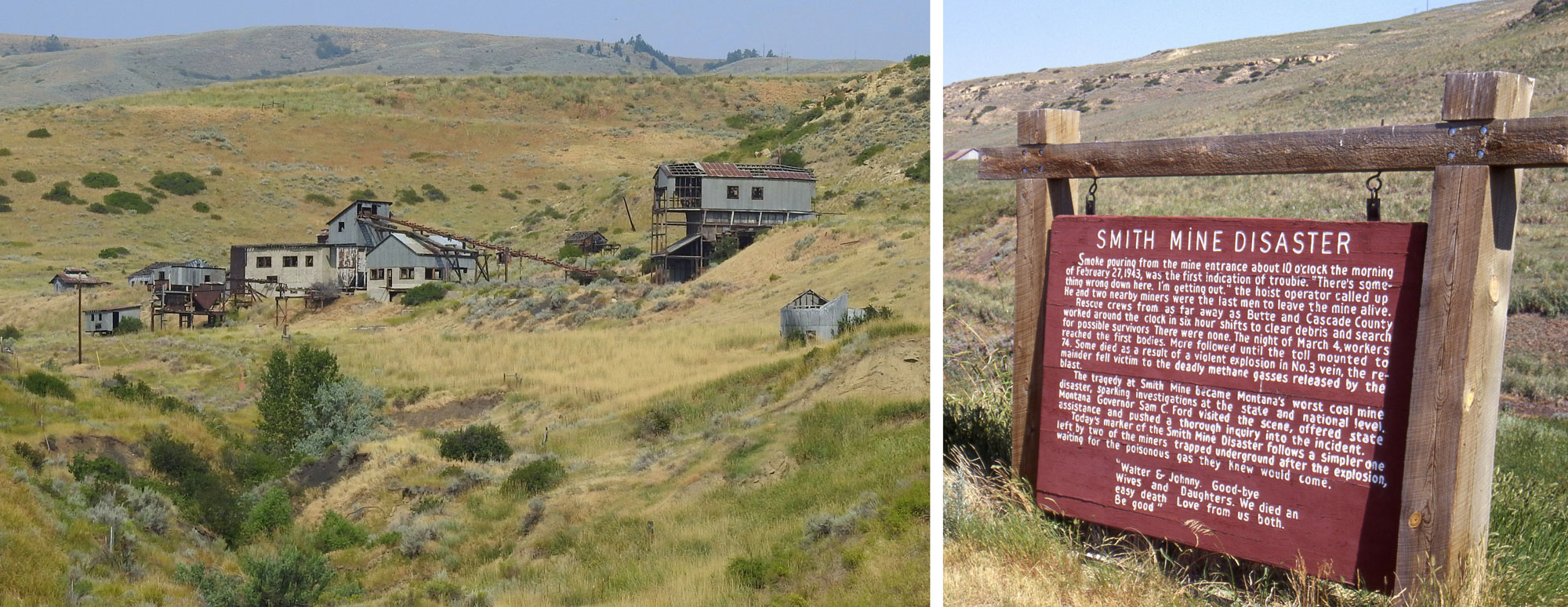
The Smith Mine in Montana was one of many coal mines where a mining disaster occurred during the 20th century in the US. The Smith Mining disaster happened on February 27, 1943, when methane in the mine ignited, causing an explosion. Ultimately, 74 men died from injuries caused by the explosion or suffocation due to toxic gases in the mine. Left: The abandoned mine. Photo by Tumbleweed 1954 (Wikimedia Commons, Creative Commons Attribution-Share Alike 4.0 International license, image cropped and resized). Right: Informational sign about the Smith Mine Disaster. Photo by Velela (Wikimedia Commons, public domain).
Methods have been developed to trap coalbed methane so that it can be used as an energy source. Water saturates fractures in some coal seams, making these seams aquifers. (An aquifer is a water-bearing, permeable rock formation that is capable of providing water in usable amounts to springs or wells.) If there is sufficient water pressure in a coal seam aquifer, methane within the coal fractures may be trapped in the coal. To extract coalbed methane, water is removed from the coal using a well. Removing water reduces the water pressure in the coal, allowing the trapped methane to escape. The gas moves out of the coal towards areas of lower pressure. As the methane moves into the well, it is separated from the water and captured.
After water is removed from a coal seam aquifer, it may take years for the aquifer to recharge (refill with water). The water in the aquifer will be slowly replenished by rain that falls at the surface and gradually works its way below the surface and to the aquifer.
Production rates for coalbed methane climbed steeply beginning in the early 1990s, and peaked in about 2008, when about a tenth of the country's yearly natural gas production came from coalbed methane. In recent years, it has declined as shale gas methane production has increased. Coalbed methane still accounts for over 5% of US methane production.
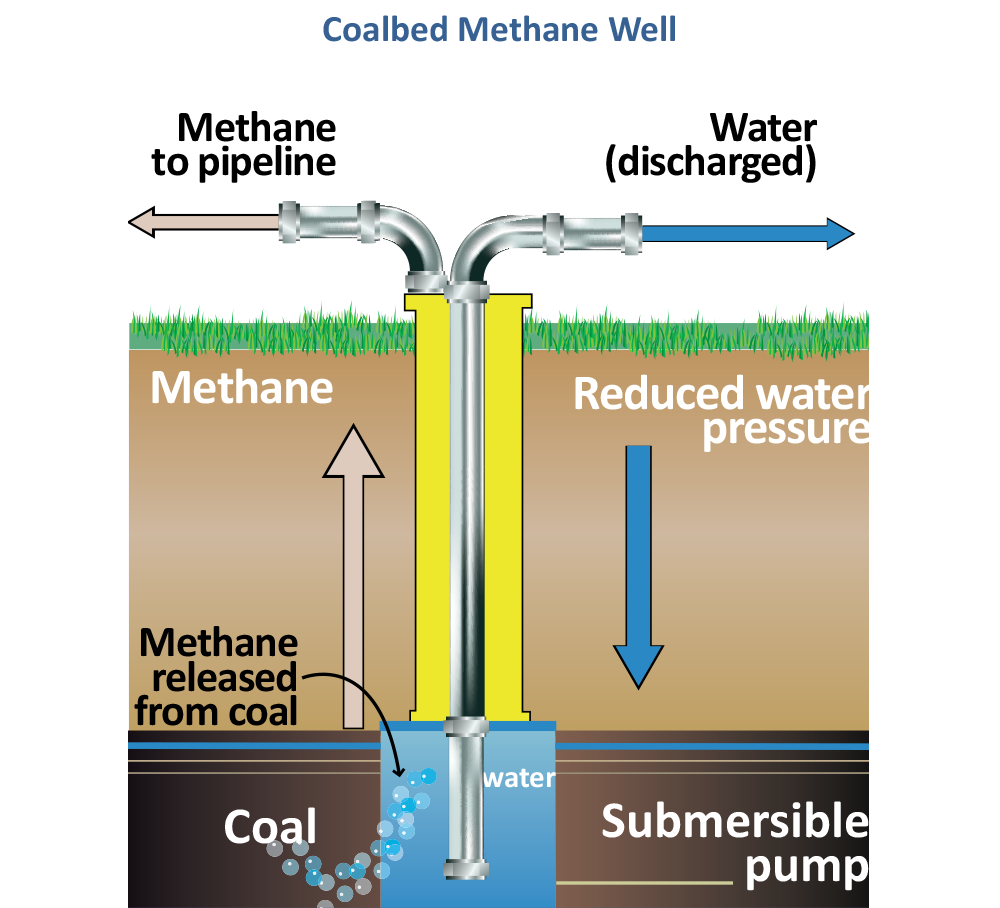
The use of a well to relieve water pressure in a coal seam, allowing the methane to escape. As water is pumped out of the coal seam, the water pressure is lowered, allowing gas to escape. The gas is captured in a separate pipe as in bubbles up in the water at the bottom of the well. Diagram from "Fossil energy research benefits: Coalbed methane" US Department of Energy Office of Fossil Energy.
Renewable energy
Renewable energy is obtained from sources that are virtually inexhaustible and that replenish over small time scales relative to human life spans. Examples of renewable energy are biomass, geothermal, hydroelectric, solar, and wind. Several of these sources are covered in more detail below:
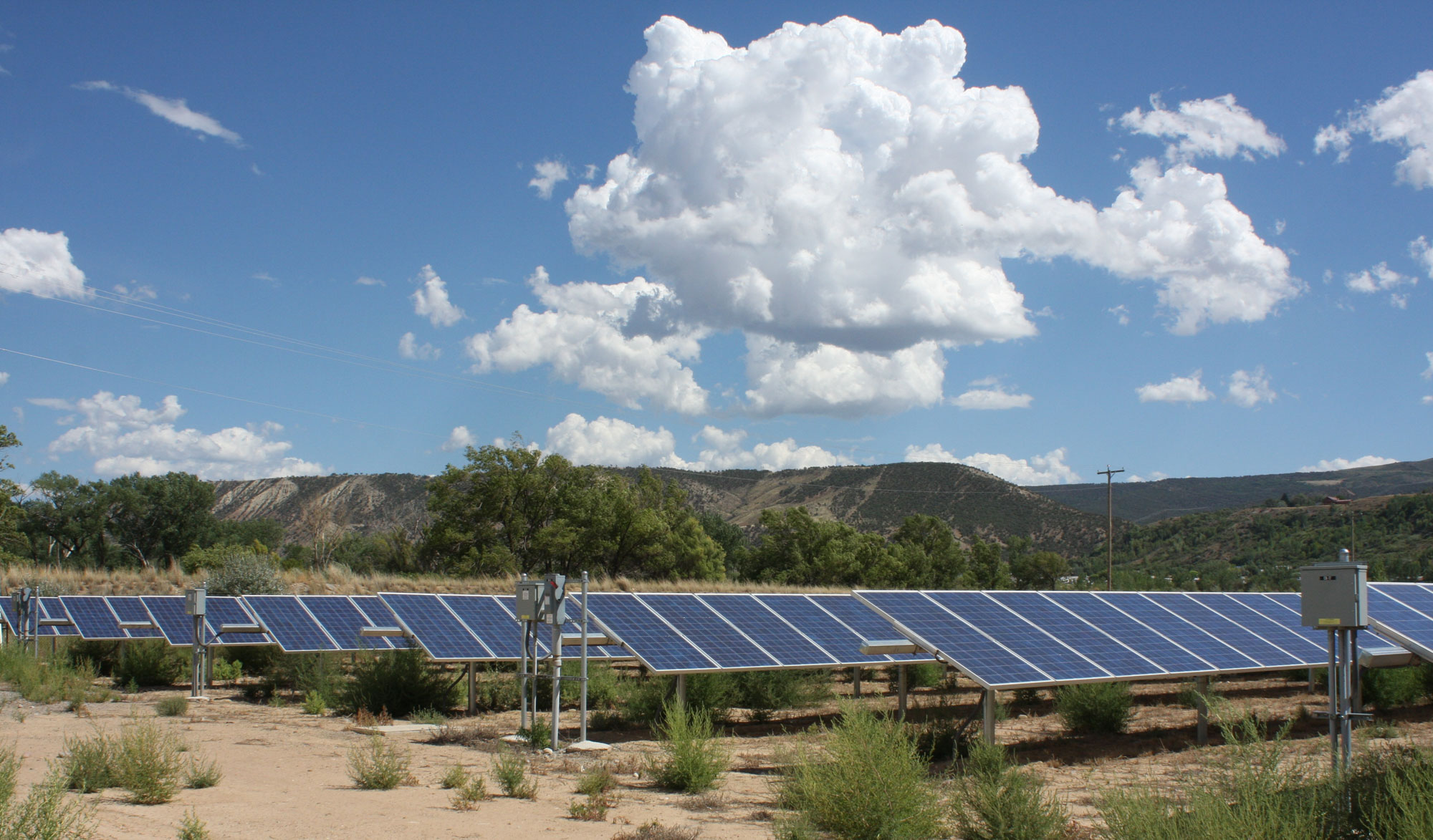
Solar panels, Colorado. Photo by Jessica K. Robertson, USGS (public domain).
Bioethanol and biomass plants
Biomass resources are organic materials that are burned to generate energy. Areas such as forestry, agriculture, and urban waste management generate hundreds of thousands of tons of biomass materials. These include oils that come from plants (soybeans and canola), as well as biomass from sugar production (sugarcane, sugar beets, and sorghum), starchy crops (grains like rice and corn), wood and wood byproducts, and certain types of municipal waste.
Geothermal energy
Geothermal energy comes from heat within the Earth, which is created on an ongoing basis by radioactivity. This energy powers mantle convection and plate tectonics. The highest-temperature conditions exist in tectonically active areas, like the Basin and Range of the western US, Iceland (part of the mid-Atlantic ridge), Japan (an area of subduction), and Hawaii and Yellowstone (areas with hot spots).
Geothermal power stations use steam to power turbines that generate electricity. The steam is created either by tapping a source of heated groundwater or by injecting water deep into the Earth where it is heated to boiling. Pressurized steam is then piped back up to the power plant, where its force turns a turbine and generates power. Water that cycles through the power plant is injected back into the underground reservoir to preserve the resource.
There are three geothermal sources that can be used to create electricity. Geopressurized or dry steam power plants utilize an existing heated groundwater source, generally around 177°C (350°F) in temperature. Petrothermal or flash steam power plants are the most common type of geothermal plant in operation today, and they actively inject water to create steam. Binary cycle power plants are able to use a lower temperature geothermal reservoir by using the warm water to heat a liquid with a lower boiling point, such as butane. The butane becomes steam, which is used to power the turbine.
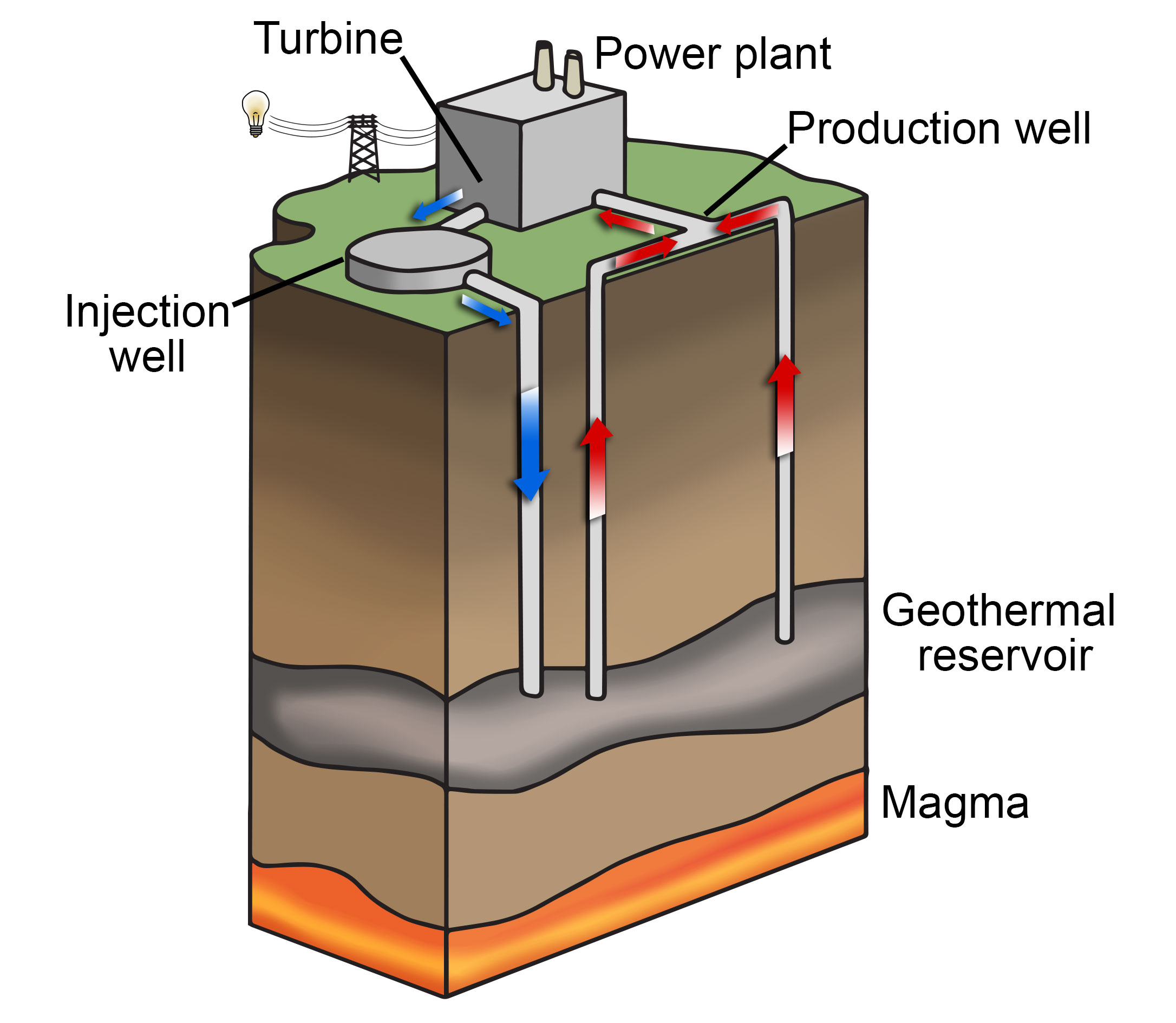
Diagram of a geothermal plant. Image modified from original by Wade Greenberg-Brand, published in The Teacher-Friendly Guide to the Geology of the Northwest Central US, edited by Mark D. Lucas, Robert M. Ross, and Andrielle N. Swaby (published by the Paleontological Research Institution, 2015) (CC BY-NC-SA 4.0 license).
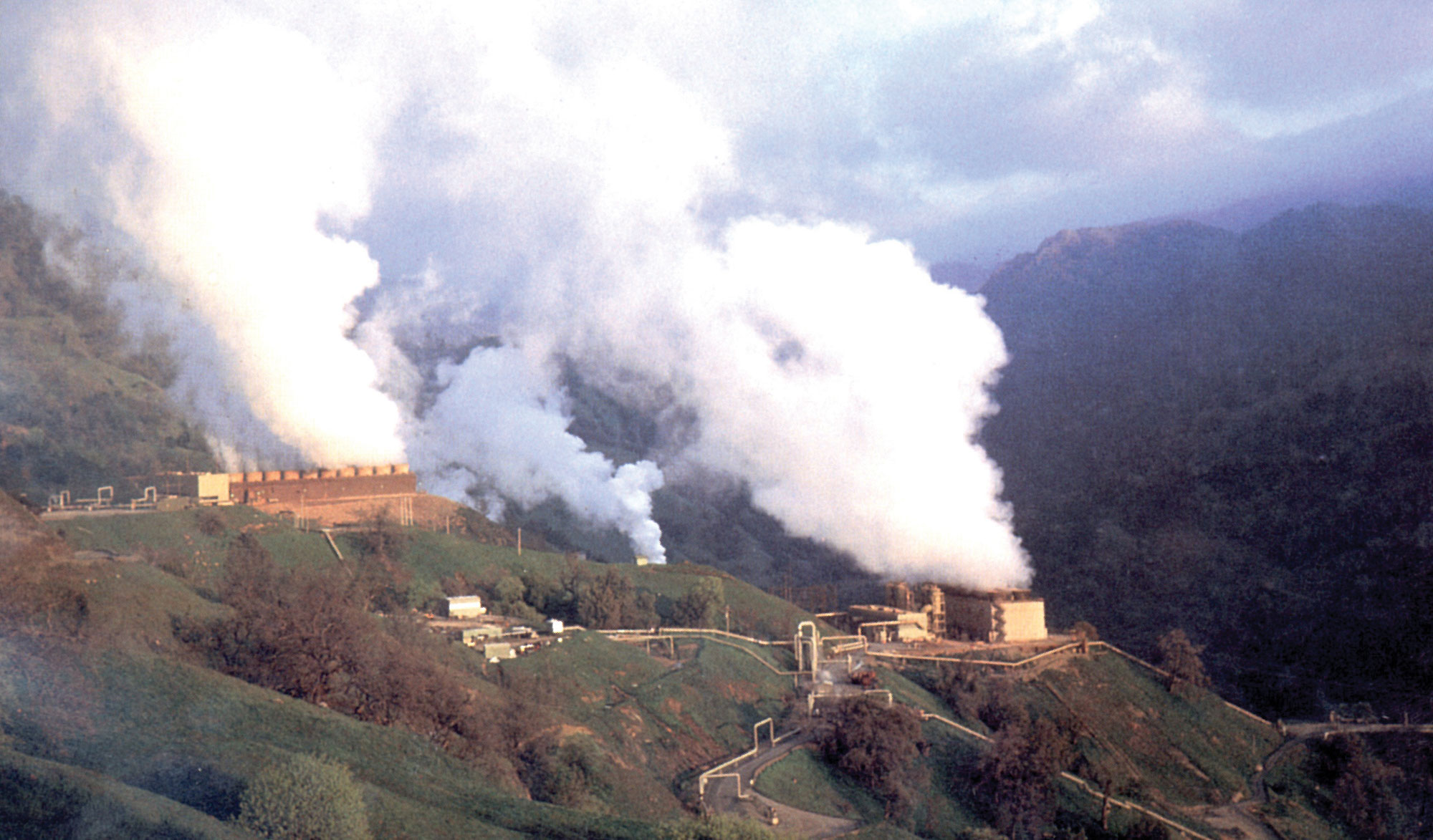
Geothermal plant, Santa Rosa, California. Photo by Julie Donnelly-Nolan, USGS (public domain).
Hydroelectricity
Hydroelectricity uses the gravitational force of falling or rushing water to rotate turbines that convert the water’s force into energy. Generating hydroelectric power requires the building of dams.
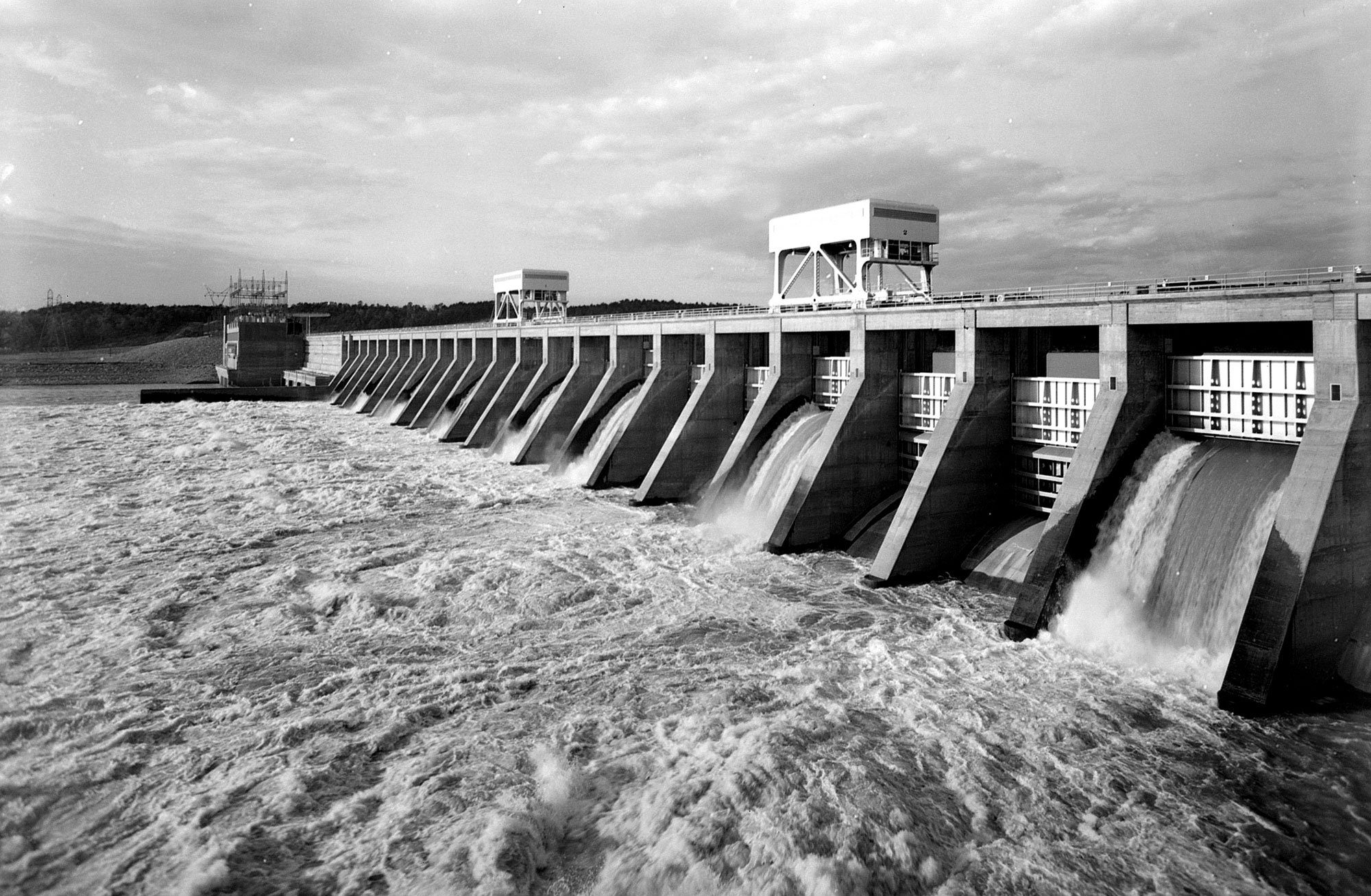
Pickwick Landing Dam on the Tennessee River, Hardin County, Tennessee, 1939. Photo by the Tennessee Valley Authority (K-1850, flickr, Creative Commons Attribution 2.0 Generic license, image resized).
Wind energy
Economically useful wind energy depends on steady high winds. Variation in wind speed is in large part influenced by the shape and elevation of the land surface. For example, higher elevations tend to have higher wind speeds, and flat areas can allow winds to pick up speed without interruption; thus high plateaus are especially appropriate for large wind farms. Since plateaus with low grass or no vegetation (or water bodies) have less wind friction than do areas of land with higher crops or forests, they facilitate higher winds.
Some regions may have locally high wind speeds that can support strategically placed wind farms. Constricted valleys parallel to wind flow may funnel air into high velocities. Elevated ridges perpendicular to wind flow can also force fast winds across them. Thus, the wind velocities of these areas can vary geographically in quite complicated ways.
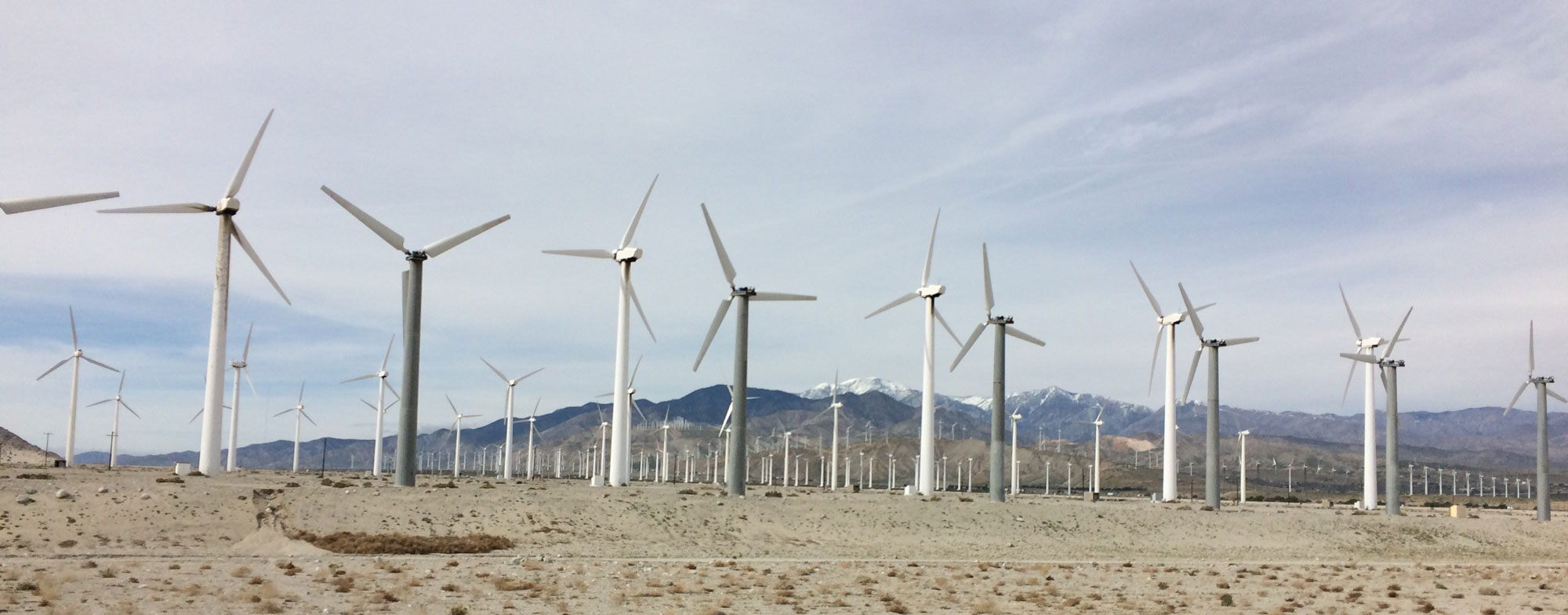
Wind turbines in California. Photo by Manuela Huso, USGS (public domain).
The future of energy in the US
Americans have come to rely on a diverse and abundant energy system, one that provides a continuous supply of energy with few interruptions. However, climate change is projected to play a big part in altering our supply, production, and demand for energy. Increases in temperatures will be accompanied by an increase in the need for energy for cooling. At the same time, projected increases in the number of severe weather events—hurricanes, floods, tornados, winter storms, and other extreme weather—will continue to have a significant effect on energy infrastructure like power grids.
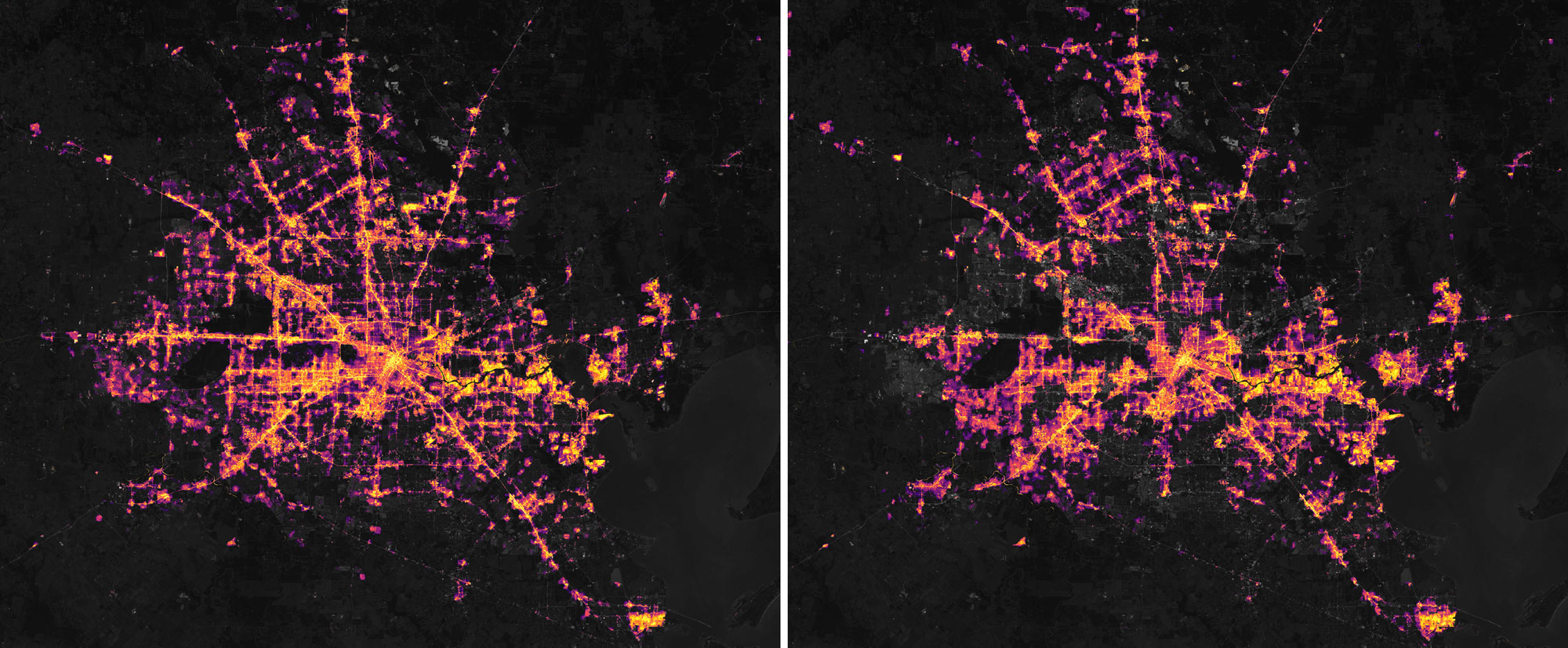
When severe winter storms hit Texas in early 2021, the state's power grid was overwhelmed by high energy demand combined with a lack of engineering for winter conditions and isolation from the power grid in the rest of the US. Power to many buildings was purposely shut off to keep the grid from failing. An estimated 1.4 million customers lost power in Houston alone. The photos above show nighttime lights in Houston before the storms (February 7, left) and following the storms (February 16, right). Photos from NASA Earth Observatory ("Extreme winter weather causes U.S. blackouts," February 17, 2021).
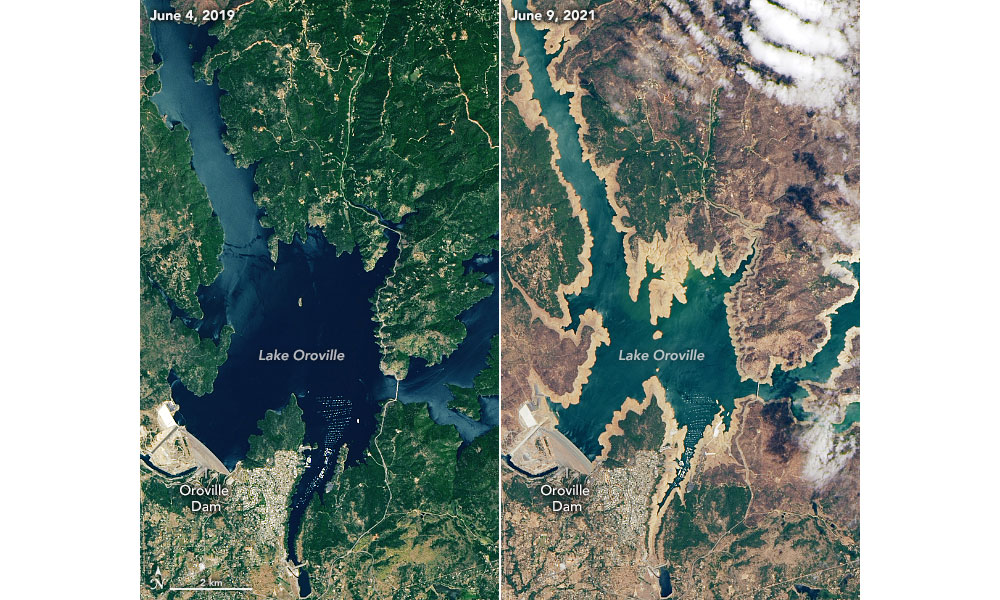
Satellite images of Lake Oroville, a reservoir in northern California. Left: At capacity (highest level), June 2019. Right: At a much lower water level due to drought, June 2021. In August 2021, the Edward Hyatt Power Plant, a hydroelectric plant on Lake Oroville, was shut down because water levels were too low. Source: USGS (public domain).
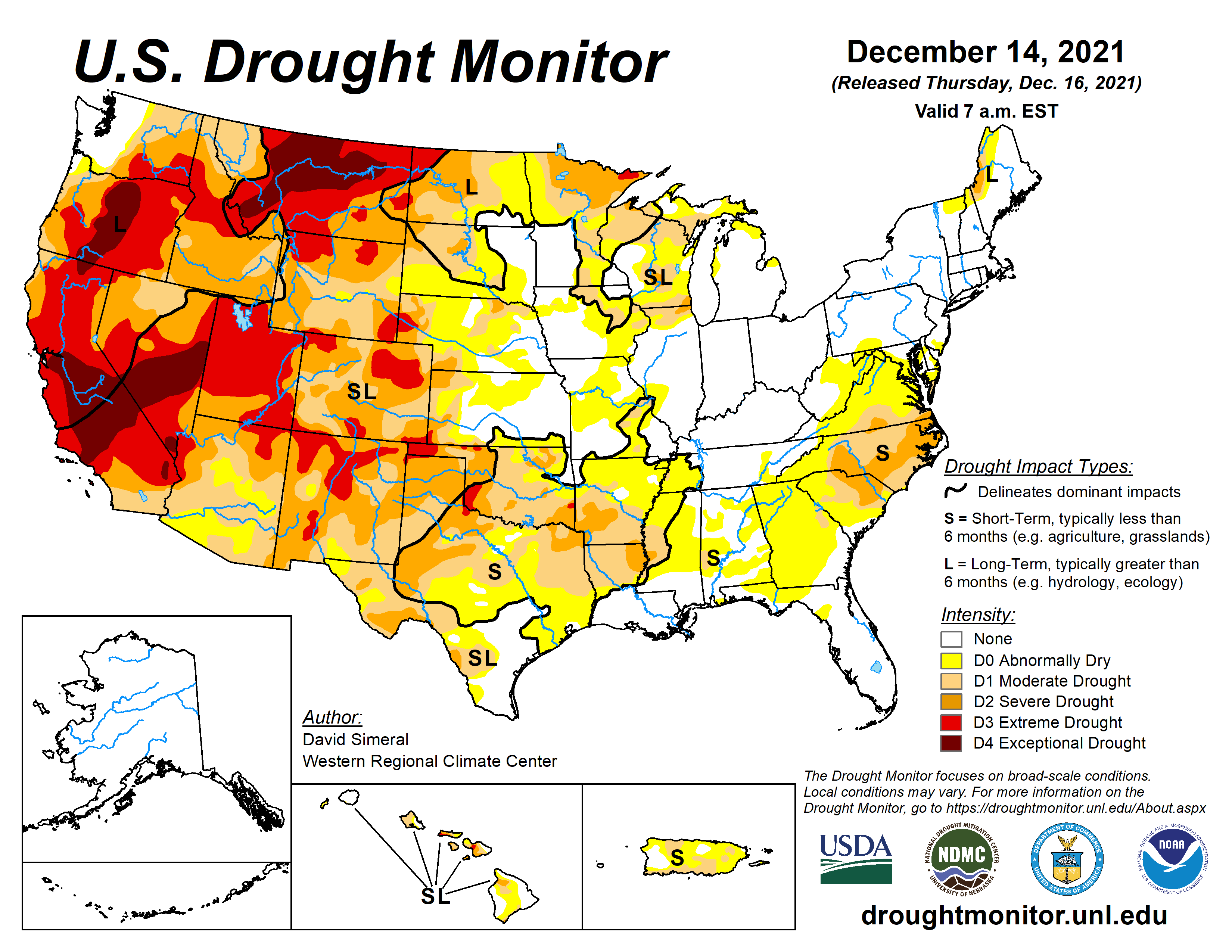
Drought conditions in the US, December 2021. Source: David Simeral, US Drought Monitor Mitigation Center at the University of Nebraska, Lincoln.
Energy is a commodity, and supply and demand around the world will also affect the US energy system. As the global population grows and industrialization of the world continues, demand for energy will increase even further as resources are depleted. These factors can significantly affect US energy costs through competition for imported and exported energy products.
Mediation of our energy production could have a huge positive impact on climate change. Unfortunately, there is no energy production system or source currently available that is truly sustainable. All forms of energy have negative impacts on the environment, as do many of the ways in which we use them.
Until we have a sustainable means of producing and delivering energy, we are faced with a sort of "energy triage"; we need to consider which means of energy production and transport make the least impact. The answer to this problem will be multifaceted, depending in large part on which energy resources and delivery methods are available in each part of the US.
Adaptation
Adaptation—changing our habits of energy use and delivery—will make it easier for our existing energy infrastructure to adjust to the needs brought on by climate change. Investing in adaptation can pay off in the short term by reducing risks and vulnerabilities, thus minimizing future risks. Increasing sustainable energy practices—including extraction, production, and usage—and improving infrastructure and delivery methods will go a long way toward decreasing the effects of climate change and increasing our energy security.
Some types of adaptation are grounded in the development of new technologies for energy production and energy efficiency, while others may be related to changes in behavior. Changes in technology and behavior may go hand in hand; roughly 2% of electricity production now goes to data centers, for example, a use that did not exist in 1985. Additionally, the internet is rapidly changing other ways in which we use energy, allowing us to telecommute and changing the way we shop.
Key points to keep in mind regarding the future of energy
- Extreme weather events are affecting energy production and delivery facilities, causing supply disruptions of varying lengths and magnitudes and affecting other infrastructure that depends on energy supply. The frequency and intensity of extreme weather events are expected to increase.
- Higher summer temperatures are likely to increase electricity use, causing higher summer peak loads, while warmer winters are likely to decrease energy demands for heating. Net energy use is projected to increase as rising demands for cooling outpace declining heating energy demands.
- Both episodic and long-lasting changes in water availability will constrain different forms of energy production.
- In the longer term, sea level rise will affect the coastal facilities and infrastructure on which many energy systems, markets, and consumers depend.
- As we invest in new energy technologies, future energy systems will differ from those of the present in uncertain ways. Depending on the ways in which our energy system changes, climate change will introduce both new risks and new opportunities.
Resources
Resources from the Paleontological Research Institution
Duggan-Haas, D., R. M. Ross, and W. D. Allmon. 2013. The science beneath the surface: A very short guide to the Marcellus Shale. Paleontological Research Institution Special Publication 43, Ithaca, NY, 252 pp. PDF: https://www.priweb.org/science-education-programs-and-resources/the-marcellus-shale-the-science-beneath-the-surface